ENCYCLOPEDIC ENTRY
Photosynthesis.
Photosynthesis is the process by which plants use sunlight, water, and carbon dioxide to create oxygen and energy in the form of sugar.

Loading ...
Learning materials, instructional links.
- Photosynthesis (Google doc)
Most life on Earth depends on photosynthesis .The process is carried out by plants, algae, and some types of bacteria, which capture energy from sunlight to produce oxygen (O 2 ) and chemical energy stored in glucose (a sugar). Herbivores then obtain this energy by eating plants, and carnivores obtain it by eating herbivores.
The process
During photosynthesis, plants take in carbon dioxide (CO 2 ) and water (H 2 O) from the air and soil. Within the plant cell, the water is oxidized, meaning it loses electrons, while the carbon dioxide is reduced, meaning it gains electrons. This transforms the water into oxygen and the carbon dioxide into glucose. The plant then releases the oxygen back into the air, and stores energy within the glucose molecules.
Chlorophyll
Inside the plant cell are small organelles called chloroplasts , which store the energy of sunlight. Within the thylakoid membranes of the chloroplast is a light-absorbing pigment called chlorophyll , which is responsible for giving the plant its green color. During photosynthesis , chlorophyll absorbs energy from blue- and red-light waves, and reflects green-light waves, making the plant appear green.
Light-dependent Reactions vs. Light-independent Reactions
While there are many steps behind the process of photosynthesis, it can be broken down into two major stages: light-dependent reactions and light-independent reactions. The light-dependent reaction takes place within the thylakoid membrane and requires a steady stream of sunlight, hence the name light- dependent reaction. The chlorophyll absorbs energy from the light waves, which is converted into chemical energy in the form of the molecules ATP and NADPH . The light-independent stage, also known as the Calvin cycle , takes place in the stroma , the space between the thylakoid membranes and the chloroplast membranes, and does not require light, hence the name light- independent reaction. During this stage, energy from the ATP and NADPH molecules is used to assemble carbohydrate molecules, like glucose, from carbon dioxide.
C3 and C4 Photosynthesis
Not all forms of photosynthesis are created equal, however. There are different types of photosynthesis, including C3 photosynthesis and C4 photosynthesis. C3 photosynthesis is used by the majority of plants. It involves producing a three-carbon compound called 3-phosphoglyceric acid during the Calvin Cycle, which goes on to become glucose. C4 photosynthesis, on the other hand, produces a four-carbon intermediate compound, which splits into carbon dioxide and a three-carbon compound during the Calvin Cycle. A benefit of C4 photosynthesis is that by producing higher levels of carbon, it allows plants to thrive in environments without much light or water. The National Geographic Society is making this content available under a Creative Commons CC-BY-NC-SA license . The License excludes the National Geographic Logo (meaning the words National Geographic + the Yellow Border Logo) and any images that are included as part of each content piece. For clarity the Logo and images may not be removed, altered, or changed in any way.
Media Credits
The audio, illustrations, photos, and videos are credited beneath the media asset, except for promotional images, which generally link to another page that contains the media credit. The Rights Holder for media is the person or group credited.
Production Managers
Program specialists, last updated.
March 20, 2024
User Permissions
For information on user permissions, please read our Terms of Service. If you have questions about how to cite anything on our website in your project or classroom presentation, please contact your teacher. They will best know the preferred format. When you reach out to them, you will need the page title, URL, and the date you accessed the resource.
If a media asset is downloadable, a download button appears in the corner of the media viewer. If no button appears, you cannot download or save the media.
Text on this page is printable and can be used according to our Terms of Service .
Interactives
Any interactives on this page can only be played while you are visiting our website. You cannot download interactives.
Related Resources

- Next Article
Cover Image

- PDF Icon PDF Link Table of Contents
- PDF Icon PDF Link Editorial Board
An overview of photosynthesis
How the photosystems work, other electron transfer chain components, abbreviations, competing interests, recommended reading and key publications, photosynthesis.
- Split-Screen
- Article contents
- Figures & tables
- Supplementary Data
- Peer Review
- Open the PDF for in another window
- Cite Icon Cite
- Get Permissions
Matthew P. Johnson; Photosynthesis. Essays Biochem 31 October 2016; 60 (3): 255–273. doi: https://doi.org/10.1042/EBC20160016
Download citation file:
- Ris (Zotero)
- Reference Manager
Photosynthesis sustains virtually all life on planet Earth providing the oxygen we breathe and the food we eat; it forms the basis of global food chains and meets the majority of humankind's current energy needs through fossilized photosynthetic fuels. The process of photosynthesis in plants is based on two reactions that are carried out by separate parts of the chloroplast. The light reactions occur in the chloroplast thylakoid membrane and involve the splitting of water into oxygen, protons and electrons. The protons and electrons are then transferred through the thylakoid membrane to create the energy storage molecules adenosine triphosphate (ATP) and nicotinomide–adenine dinucleotide phosphate (NADPH). The ATP and NADPH are then utilized by the enzymes of the Calvin–Benson cycle (the dark reactions), which converts CO 2 into carbohydrate in the chloroplast stroma. The basic principles of solar energy capture, energy, electron and proton transfer and the biochemical basis of carbon fixation are explained and their significance is discussed.
Introduction
Photosynthesis is the ultimate source of all of humankind's food and oxygen, whereas fossilized photosynthetic fuels provide ∼87% of the world's energy. It is the biochemical process that sustains the biosphere as the basis for the food chain. The oxygen produced as a by-product of photosynthesis allowed the formation of the ozone layer, the evolution of aerobic respiration and thus complex multicellular life.
Oxygenic photosynthesis involves the conversion of water and CO 2 into complex organic molecules such as carbohydrates and oxygen. Photosynthesis may be split into the ‘light’ and ‘dark’ reactions. In the light reactions, water is split using light into oxygen, protons and electrons, and in the dark reactions, the protons and electrons are used to reduce CO 2 to carbohydrate (given here by the general formula CH 2 O). The two processes can be summarized thus:
Light reactions:

Dark reactions:

The positive sign of the standard free energy change of the reaction (Δ G °) given above means that the reaction requires energy ( an endergonic reaction ). The energy required is provided by absorbed solar energy, which is converted into the chemical bond energy of the products ( Box 1 ).

Photosynthesis converts ∼200 billion tonnes of CO 2 into complex organic compounds annually and produces ∼140 billion tonnes of oxygen into the atmosphere. By facilitating conversion of solar energy into chemical energy, photosynthesis acts as the primary energy input into the global food chain. Nearly all living organisms use the complex organic compounds derived from photosynthesis as a source of energy. The breakdown of these organic compounds occurs via the process of aerobic respiration, which of course also requires the oxygen produced by photosynthesis.

Unlike photosynthesis, aerobic respiration is an exergonic process (negative Δ G °) with the energy released being used by the organism to power biosynthetic processes that allow growth and renewal, mechanical work (such as muscle contraction or flagella rotation) and facilitating changes in chemical concentrations within the cell (e.g. accumulation of nutrients and expulsion of waste). The use of exergonic reactions to power endergonic ones associated with biosynthesis and housekeeping in biological organisms such that the overall free energy change is negative is known as ‘ coupling’.
Photosynthesis and respiration are thus seemingly the reverse of one another, with the important caveat that both oxygen formation during photosynthesis and its utilization during respiration result in its liberation or incorporation respectively into water rather than CO 2 . In addition, glucose is one of several possible products of photosynthesis with amino acids and lipids also being synthesized rapidly from the primary photosynthetic products.
The consideration of photosynthesis and respiration as opposing processes helps us to appreciate their role in shaping our environment. The fixation of CO 2 by photosynthesis and its release during breakdown of organic molecules during respiration, decay and combustion of organic matter and fossil fuels can be visualized as the global carbon cycle ( Figure 1 ).
The global carbon cycle

The relationship between respiration, photosynthesis and global CO 2 and O 2 levels.
At present, this cycle may be considered to be in a state of imbalance due to the burning of fossil fuels (fossilized photosynthesis), which is increasing the proportion of CO 2 entering the Earth's atmosphere, leading to the so-called ‘greenhouse effect’ and human-made climate change.
Oxygenic photosynthesis is thought to have evolved only once during Earth's history in the cyanobacteria. All other organisms, such as plants, algae and diatoms, which perform oxygenic photosynthesis actually do so via cyanobacterial endosymbionts or ‘chloroplasts’. An endosymbiotoic event between an ancestral eukaryotic cell and a cyanobacterium that gave rise to plants is estimated to have occurred ∼1.5 billion years ago. Free-living cyanobacteria still exist today and are responsible for ∼50% of the world's photosynthesis. Cyanobacteria themselves are thought to have evolved from simpler photosynthetic bacteria that use either organic or inorganic compounds such a hydrogen sulfide as a source of electrons rather than water and thus do not produce oxygen.
The site of photosynthesis in plants
In land plants, the principal organs of photosynthesis are the leaves ( Figure 2 A). Leaves have evolved to expose the largest possible area of green tissue to light and entry of CO 2 to the leaf is controlled by small holes in the lower epidermis called stomata ( Figure 2 B). The size of the stomatal openings is variable and regulated by a pair of guard cells, which respond to the turgor pressure (water content) of the leaf, thus when the leaf is hydrated, the stomata can open to allow CO 2 in. In contrast, when water is scarce, the guard cells lose turgor pressure and close, preventing the escape of water from the leaf via transpiration.
Location of the photosynthetic machinery

( A ) The model plant Arabidopsis thaliana . ( B ) Basic structure of a leaf shown in cross-section. Chloroplasts are shown as green dots within the cells. ( C ) An electron micrograph of an Arabidopsis chloroplast within the leaf. ( D ) Close-up region of the chloroplast showing the stacked structure of the thylakoid membrane.
Within the green tissue of the leaf (mainly the mesophyll) each cell (∼100 μm in length) contains ∼100 chloroplasts (2–3 μm in length), the tiny organelles where photosynthesis takes place. The chloroplast has a complex structure ( Figure 2 C, D) with two outer membranes (the envelope), which are colourless and do not participate in photosynthesis, enclosing an aqueous space (the stroma) wherein sits a third membrane known as the thylakoid, which in turn encloses a single continuous aqueous space called the lumen.
The light reactions of photosynthesis involve light-driven electron and proton transfers, which occur in the thylakoid membrane, whereas the dark reactions involve the fixation of CO 2 into carbohydrate, via the Calvin–Benson cycle, which occurs in the stroma ( Figure 3 ). The light reactions involve electron transfer from water to NADP + to form NADPH and these reactions are coupled to proton transfers that lead to the phosphorylation of adenosine diphosphate (ADP) into ATP. The Calvin–Benson cycle uses ATP and NADPH to convert CO 2 into carbohydrates ( Figure 3 ), regenerating ADP and NADP + . The light and dark reactions are therefore mutually dependent on one another.
Division of labour within the chloroplast

The light reactions of photosynthesis take place in the thylakoid membrane, whereas the dark reactions are located in the chloroplast stroma.
Photosynthetic electron and proton transfer chain
The light-driven electron transfer reactions of photosynthesis begin with the splitting of water by Photosystem II (PSII). PSII is a chlorophyll–protein complex embedded in the thylakoid membrane that uses light to oxidize water to oxygen and reduce the electron acceptor plastoquinone to plastoquinol. Plastoquinol in turn carries the electrons derived from water to another thylakoid-embedded protein complex called cytochrome b 6 f (cyt b 6 f ). cyt b 6 f oxidizes plastoquinol to plastoquinone and reduces a small water-soluble electron carrier protein plastocyanin, which resides in the lumen. A second light-driven reaction is then carried out by another chlorophyll protein complex called Photosystem I (PSI). PSI oxidizes plastocyanin and reduces another soluble electron carrier protein ferredoxin that resides in the stroma. Ferredoxin can then be used by the ferredoxin–NADP + reductase (FNR) enzyme to reduce NADP + to NADPH. This scheme is known as the linear electron transfer pathway or Z-scheme ( Figure 4 ).
The photosynthetic electron and proton transfer chain

The linear electron transfer pathway from water to NADP + to form NADPH results in the formation of a proton gradient across the thylakoid membrane that is used by the ATP synthase enzyme to make ATP.
The Z-scheme, so-called since it resembles the letter ‘Z’ when turned on its side ( Figure 5 ), thus shows how the electrons move from the water–oxygen couple (+820 mV) via a chain of redox carriers to NADP + /NADPH (−320 mV) during photosynthetic electron transfer. Generally, electrons are transferred from redox couples with low potentials (good reductants) to those with higher potentials (good oxidants) (e.g. during respiratory electron transfer in mitochondria) since this process is exergonic (see Box 2 ). However, photosynthetic electron transfer also involves two endergonic steps, which occur at PSII and at PSI and require an energy input in the form of light. The light energy is used to excite an electron within a chlorophyll molecule residing in PSII or PSI to a higher energy level; this excited chlorophyll is then able to reduce the subsequent acceptors in the chain. The oxidized chlorophyll is then reduced by water in the case of PSII and plastocyanin in the case of PSI.
Z-scheme of photosynthetic electron transfer

The main components of the linear electron transfer pathway are shown on a scale of redox potential to illustrate how two separate inputs of light energy at PSI and PSII result in the endergonic transfer of electrons from water to NADP + .
The water-splitting reaction at PSII and plastoquinol oxidation at cyt b 6 f result in the release of protons into the lumen, resulting in a build-up of protons in this compartment relative to the stroma. The difference in the proton concentration between the two sides of the membrane is called a proton gradient. The proton gradient is a store of free energy (similar to a gradient of ions in a battery) that is utilized by a molecular mechanical motor ATP synthase, which resides in the thylakoid membrane ( Figure 4 ). The ATP synthase allows the protons to move down their concentration gradient from the lumen (high H + concentration) to the stroma (low H + concentration). This exergonic reaction is used to power the endergonic synthesis of ATP from ADP and inorganic phosphate (P i ). This process of photophosphorylation is thus essentially similar to oxidative phosphorylation, which occurs in the inner mitochondrial membrane during respiration.
An alternative electron transfer pathway exists in plants and algae, known as cyclic electron flow. Cyclic electron flow involves the recycling of electrons from ferredoxin to plastoquinone, with the result that there is no net production of NADPH; however, since protons are still transferred into the lumen by oxidation of plastoquinol by cyt b 6 f , ATP can still be formed. Thus photosynthetic organisms can control the ratio of NADPH/ATP to meet metabolic need by controlling the relative amounts of cyclic and linear electron transfer.

Light absorption by pigments
Photosynthesis begins with the absorption of light by pigments molecules located in the thylakoid membrane. The most well-known of these is chlorophyll, but there are also carotenoids and, in cyanobacteria and some algae, bilins. These pigments all have in common within their chemical structures an alternating series of carbon single and double bonds, which form a conjugated system π–electron system ( Figure 6 ).
Major photosynthetic pigments in plants

The chemical structures of the chlorophyll and carotenoid pigments present in the thylakoid membrane. Note the presence in each of a conjugated system of carbon–carbon double bonds that is responsible for light absorption.
The variety of pigments present within each type of photosynthetic organism reflects the light environment in which it lives; plants on land contain chlorophylls a and b and carotenoids such as β-carotene, lutein, zeaxanthin, violaxanthin, antheraxanthin and neoxanthin ( Figure 6 ). The chlorophylls absorb blue and red light and so appear green in colour, whereas carotenoids absorb light only in the blue and so appear yellow/red ( Figure 7 ), colours more obvious in the autumn as chlorophyll is the first pigment to be broken down in decaying leaves.
Basic absorption spectra of the major chlorophyll and carotenoid pigments found in plants

Chlorophylls absorb light energy in the red and blue part of the visible spectrum, whereas carotenoids only absorb light in the blue/green.
Light, or electromagnetic radiation, has the properties of both a wave and a stream of particles (light quanta). Each quantum of light contains a discrete amount of energy that can be calculated by multiplying Planck's constant, h (6.626×10 −34 J·s) by ν, the frequency of the radiation in cycles per second (s −1 ):

The frequency (ν) of the light and so its energy varies with its colour, thus blue photons (∼450 nm) are more energetic than red photons (∼650 nm). The frequency (ν) and wavelength (λ) of light are related by:

where c is the velocity of light (3.0×10 8 m·s −1 ), and the energy of a particular wavelength (λ) of light is given by:

Thus 1 mol of 680 nm photons of red light has an energy of 176 kJ·mol −1 .
The electrons within the delocalized π system of the pigment have the ability to jump up from the lowest occupied molecular orbital (ground state) to higher unoccupied molecular electron orbitals (excited states) via the absorption of specific wavelengths of light in the visible range (400–725 nm). Chlorophyll has two excited states known as S 1 and S 2 and, upon interaction of the molecule with a photon of light, one of its π electrons is promoted from the ground state (S 0 ) to an excited state, a process taking just 10 −15 s ( Figure 8 ). The energy gap between the S 0 and S 1 states is spanned by the energy provided by a red photon (∼600–700 nm), whereas the energy gap between the S 0 and S 2 states is larger and therefore requires a more energetic (shorter wavelength, higher frequency) blue photon (∼400–500 nm) to span the energy gap.
Jablonski diagram of chlorophyll showing the possible fates of the S 1 and S 2 excited states and timescales of the transitions involved

Photons with slightly different energies (colours) excite each of the vibrational substates of each excited state (as shown by variation in the size and colour of the arrows).
Upon excitation, the electron in the S 2 state quickly undergoes losses of energy as heat through molecular vibration and undergoes conversion into the energy of the S 1 state by a process called internal conversion. Internal conversion occurs on a timescale of 10 −12 s. The energy of a blue photon is thus rapidly degraded to that of a red photon. Excitation of the molecule with a red photon would lead to promotion of an electron to the S 1 state directly. Once the electron resides in the S 1 state, it is lower in energy and thus stable on a somewhat longer timescale (10 −9 s). The energy of the excited electron in the S 1 state can have one of several fates: it could return to the ground state (S 0 ) by emission of the energy as a photon of light (fluorescence), or it could be lost as heat due to internal conversion between S 1 and S 0 . Alternatively, if another chlorophyll is nearby, a process known as excitation energy transfer (EET) can result in the non-radiative exchange of energy between the two molecules ( Figure 9 ). For this to occur, the two chlorophylls must be close by (<7 nm), have a specific orientation with respect to one another, and excited state energies that overlap (are resonant) with one another. If these conditions are met, the energy is exchanged, resulting in a mirror S 0 →S 1 transition in the acceptor molecule and a S 1 →S 0 transition in the other.
Basic mechanism of excitation energy transfer between chlorophyll molecules

Two chlorophyll molecules with resonant S 1 states undergo a mirror transition resulting in the non-radiative transfer of excitation energy between them.
Light-harvesting complexes
In photosynthetic systems, chlorophylls and carotenoids are found attached to membrane-embedded proteins known as light-harvesting complexes (LHCs). Through careful binding and orientation of the pigment molecules, absorbed energy can be transferred among them by EET. Each pigment is bound to the protein by a series of non-covalent bonding interactions (such as, hydrogen bonds, van der Waals interactions, hydrophobic interaction and co-ordination bonds between lone pair electrons of residues such as histidine in the protein and the Mg 2+ ion in chlorophyll); the protein structure is such that each bound pigment experiences a slightly different environment in terms of the surrounding amino acid side chains, lipids, etc., meaning that the S 1 and S 2 energy levels are shifted in energy with respect to that of other neighbouring pigment molecules. The effect is to create a range of pigment energies that act to ‘funnel’ the energy on to the lowest-energy pigments in the LHC by EET.
Reaction centres
A photosystem consists of numerous LHCs that form an antenna of hundreds of pigment molecules. The antenna pigments act to collect and concentrate excitation energy and transfer it towards a ‘special pair’ of chlorophyll molecules that reside in the reaction centre (RC) ( Figure 10 ). Unlike the antenna pigments, the special pair of chlorophylls are ‘redox-active’ in the sense that they can return to the ground state (S 0 ) by the transfer of the electron residing in the S 1 excited state (Chl*) to another species. This process is known as charge separation and result in formation of an oxidized special pair (Chl + ) and a reduced acceptor (A − ). The acceptor in PSII is plastoquinone and in PSI it is ferredoxin. If the RC is to go on functioning, the electron deficiency on the special pair must be made good, in PSII the electron donor is water and in PSI it is plastocyanin.
Basic structure of a photosystem

Light energy is captured by the antenna pigments and transferred to the special pair of RC chlorophylls which undergo a redox reaction leading to reduction of an acceptor molecule. The oxidized special pair is regenerated by an electron donor.
It is worth asking why photosynthetic organisms bother to have a large antenna of pigments serving an RC rather than more numerous RCs. The answer lies in the fact that the special pair of chlorophylls alone have a rather small spatial and spectral cross-section, meaning that there is a limit to the amount of light they can efficiently absorb. The amount of light they can practically absorb is around two orders of magnitude smaller than their maximum possible turnover rate, Thus LHCs act to increase the spatial (hundreds of pigments) and spectral (several types of pigments with different light absorption characteristics) cross-section of the RC special pair ensuring that its turnover rate runs much closer to capacity.
Photosystem II
PSII is a light-driven water–plastoquinone oxidoreductase and is the only enzyme in Nature that is capable of performing the difficult chemistry of splitting water into protons, electrons and oxygen ( Figure 11 ). In principle, water is an extremely poor electron donor since the redox potential of the water–oxygen couple is +820 mV. PSII uses light energy to excite a special pair of chlorophylls, known as P680 due to their 680 nm absorption peak in the red part of the spectrum. P680* undergoes charge separation that results in the formation of an extremely oxidizing species P680 + which has a redox potential of +1200 mV, sufficient to oxidize water. Nonetheless, since water splitting involves four electron chemistry and charge separation only involves transfer of one electron, four separate charge separations (turnovers of PSII) are required to drive formation of one molecule of O 2 from two molecules of water. The initial electron donation to generate the P680 from P680 + is therefore provided by a cluster of manganese ions within the oxygen-evolving complex (OEC), which is attached to the lumen side of PSII ( Figure 12 ). Manganese is a transition metal that can exist in a range of oxidation states from +1 to +5 and thus accumulates the positive charges derived from each light-driven turnover of P680. Progressive extraction of electrons from the manganese cluster is driven by the oxidation of P680 within PSII by light and is known as the S-state cycle ( Figure 12 ). After the fourth turnover of P680, sufficient positive charge is built up in the manganese cluster to permit the splitting of water into electrons, which regenerate the original state of the manganese cluster, protons, which are released into the lumen and contribute to the proton gradient used for ATP synthesis, and the by-product O 2 . Thus charge separation at P680 provides the thermodynamic driving force, whereas the manganese cluster acts as a catalyst for the water-splitting reaction.
Basic structure of the PSII–LHCII supercomplex from spinach

The organization of PSII and its light-harvesting antenna. Protein is shown in grey, with chlorophylls in green and carotenoids in orange. Drawn from PDB code 3JCU
S-state cycle of water oxidation by the manganese cluster (shown as circles with roman numerals representing the manganese ion oxidation states) within the PSII oxygen-evolving complex

Progressive extraction of electrons from the manganese cluster is driven by the oxidation of P680 within PSII by light. Each of the electrons given up by the cluster is eventually repaid at the S 4 to S 0 transition when molecular oxygen (O 2 ) is formed. The protons extracted from water during the process are deposited into the lumen and contribute to the protonmotive force.
The electrons yielded by P680* following charge separation are not passed directly to plastoquinone, but rather via another acceptor called pheophytin, a porphyrin molecule lacking the central magnesium ion as in chlorophyll. Plastoquinone reduction to plastoquinol requires two electrons and thus two molecules of plastoquinol are formed per O 2 molecule evolved by PSII. Two protons are also taken up upon formation of plastoquinol and these are derived from the stroma. PSII is found within the thylakoid membrane of plants as a dimeric RC complex surrounded by a peripheral antenna of six minor monomeric antenna LHC complexes and two to eight trimeric LHC complexes, which together form a PSII–LHCII supercomplex ( Figure 11 ).
Photosystem I
PSI is a light-driven plastocyanin–ferredoxin oxidoreductase ( Figure 13 ). In PSI, the special pair of chlorophylls are known as P700 due to their 700 nm absorption peak in the red part of the spectrum. P700* is an extremely strong reductant that is able to reduce ferredoxin which has a redox potential of −450 mV (and is thus is, in principle, a poor electron acceptor). Reduced ferredoxin is then used to generate NADPH for the Calvin–Benson cycle at a separate complex known as FNR. The electron from P700* is donated via another chlorophyll molecule and a bound quinone to a series of iron–sulfur clusters at the stromal side of the complex, whereupon the electron is donated to ferredoxin. The P700 species is regenerated form P700 + via donation of an electron from the soluble electron carrier protein plastocyanin.
Basic structure of the PSI–LHCI supercomplex from pea

The organization of PSI and its light-harvesting antenna. Protein is shown in grey, with chlorophylls in green and carotenoids in orange. Drawn from PDB code 4XK8.
PSI is found within the thylakoid membrane as a monomeric RC surrounded on one side by four LHC complexes known as LHCI. The PSI–LHCI supercomplex is found mainly in the unstacked regions of the thylakoid membrane ( Figure 13 ).
Plastoquinone/plastoquinol
Plastoquinone is a small lipophilic electron carrier molecule that resides within the thylakoid membrane and carries two electrons and two protons from PSII to the cyt b 6 f complex. It has a very similar structure to that of the molecule ubiquinone (coenzyme Q 10 ) in the mitochondrial inner membrane.
Cytochrome b 6 f complex
The cyt b 6 f complex is a plastoquinol–plastocyanin oxidoreductase and possess a similar structure to that of the cytochrome bc 1 complex (complex III) in mitochondria ( Figure 14 A). As with Complex III, cyt b 6 f exists as a dimer in the membrane and carries out both the oxidation and reduction of quinones via the so-called Q-cycle. The Q-cycle ( Figure 14 B) involves oxidation of one plastoquinol molecule at the Qp site of the complex, both protons from this molecule are deposited in the lumen and contribute to the proton gradient for ATP synthesis. The two electrons, however, have different fates. The first is transferred via an iron–sulfur cluster and a haem cofactor to the soluble electron carrier plastocyanin (see below). The second electron derived from plastoquinol is passed via two separate haem cofactors to another molecule of plastoquinone bound to a separate site (Qn) on the complex, thus reducing it to a semiquinone. When a second plastoquinol molecule is oxidized at Qp, a second molecule of plastocyanin is reduced and two further protons are deposited in the lumen. The second electron reduces the semiquinone at the Qn site which, concomitant with uptake of two protons from the stroma, causes its reduction to plastoquinol. Thus for each pair of plastoquinol molecules oxidized by the complex, one is regenerated, yet all four protons are deposited into the lumen. The Q-cycle thus doubles the number of protons transferred from the stroma to the lumen per plastoquinol molecule oxidized.

( A ) Structure drawn from PDB code 1Q90. ( B ) The protonmotive Q-cycle showing how electrons from plastoquinol are passed to both plastocyanin and plastoquinone, doubling the protons deposited in the lumen for every plastoquinol molecule oxidized by the complex.
Plastocyanin
Plastocyanin is a small soluble electron carrier protein that resides in the thylakoid lumen. The active site of the plastocyanin protein binds a copper ion, which cycles between the Cu 2+ and Cu + oxidation states following its oxidation by PSI and reduction by cyt b 6 f respectively.
Ferredoxin is a small soluble electron carrier protein that resides in the chloroplast stroma. The active site of the ferredoxin protein binds an iron–sulfur cluster, which cycles between the Fe 2+ and Fe 3+ oxidation states following its reduction by PSI and oxidation by the FNR complex respectively.
Ferredoxin–NADP + reductase
The FNR complex is found in both soluble and thylakoid membrane-bound forms. The complex binds a flavin–adenine dinucleotide (FAD) cofactor at its active site, which accepts two electrons from two molecules of ferredoxin before using them reduce NADP + to NADPH.
ATP synthase
The ATP synthase enzyme is responsible for making ATP from ADP and P i ; this endergonic reaction is powered by the energy contained within the protonmotive force. According to the structure, 4.67 H + are required for every ATP molecule synthesized by the chloroplast ATP synthase. The enzyme is a rotary motor which contains two domains: the membrane-spanning F O portion which conducts protons from the lumen to the stroma, and the F 1 catalytic domain that couples this exergonic proton movement to ATP synthesis.
Membrane stacking and the regulation of photosynthesis
Within the thylakoid membrane, PSII–LHCII supercomplexes are packed together into domains known as the grana, which associate with one another to form grana stacks. PSI and ATP synthase are excluded from these stacked PSII–LHCII regions by steric constraints and thus PSII and PSI are segregated in the thylakoid membrane between the stacked and unstacked regions ( Figure 15 ). The cyt b 6 f complex, in contrast, is evenly distributed throughout the grana and stromal lamellae. The evolutionary advantage of membrane stacking is believed to be a higher efficiency of electron transport by preventing the fast energy trap PSI from ‘stealing’ excitation energy from the slower trap PSII, a phenomenon known as spillover. Another possible advantage of membrane stacking in thylakoids may be the segregation of the linear and cyclic electron transfer pathways, which might otherwise compete to reduce plastoquinone. In this view, PSII, cyt b 6 f and a sub-fraction of PSI closest to the grana is involved in linear flow, whereas PSI and cyt b 6 f in the stromal lamellae participates in cyclic flow. The cyclic electron transfer pathway recycles electrons from ferredoxin back to plastoquinone and thus allows protonmotive force generation (and ATP synthesis) without net NADPH production. Cyclic electron transfer thereby provides the additional ATP required for the Calvin–Benson cycle (see below).
Lateral heterogeneity in thylakoid membrane organization

( A ) Electron micrograph of the thylakoid membrane showing stacked grana and unstacked stromal lamellae regions. ( B ) Model showing the distribution of the major complexes of photosynthetic electron and proton transfer between the stacked grana and unstacked stromal lamellae regions.
‘Dark’ reactions: the Calvin–Benson cycle
CO 2 is fixed into carbohydrate via the Calvin–Benson cycle in plants, which consumes the ATP and NADPH produced during the light reactions and thus in turn regenerates ADP, P i and NADP + . In the first step of the Calvin–Benson cycle ( Figure 16 ), CO 2 is combined with a 5-carbon (5C) sugar, ribulose 1,5-bisphosphate in a reaction catalysed by the enzyme ribulose-1,5-bisphosphate carboxylase/oxygenase (Rubisco). The reaction forms an unstable 6C intermediate that immediately splits into two molecules of 3-phosphoglycerate. 3-Phosphoglycerate is first phosphorylated by 3-phosphoglycerate kinase using ATP to form 1,3-bisphosphoglycerate. 1,3-Bisphosphoglycerate is then reduced by glyceraldehyde 3-phosphate dehydrogenase using NADPH to form glyceraldehyde 3-phosphate (GAP, a triose or 3C sugar) in reactions, which are the reverse of glycolysis. For every three CO 2 molecules initially combined with ribulose 1,5-bisphopshate, six molecules of GAP are produced by the subsequent steps. However only one of these six molecules can be considered as a product of the Calvin–Benson cycle since the remaining five are required to regenerate ribulose 1,5-bisphosphate in a complex series of reactions that also require ATP. The one molecule of GAP that is produced for each turn of the cycle can be quickly converted by a range of metabolic pathways into amino acids, lipids or sugars such as glucose. Glucose in turn may be stored as the polymer starch as large granules within chloroplasts.
The Calvin–Benson cycle

Overview of the biochemical pathway for the fixation of CO 2 into carbohydrate in plants.
A complex biochemical ‘dance’ ( Figure 16 ) is then involved in the regeneration of three ribulose 1,5-bisphosphate (5C) from the remaining five GAP (3C) molecules. The regeneration begins with the conversion of two molecules of GAP into dihydroxyacetone phosphate (DHAP) by triose phosphate isomerase; one of the DHAP molecules is the combined with another GAP molecule to make fructose 1,6-bisphosphate (6C) by aldolase. The fructose 1,6-bisphosphate is then dephosphorylated by fructose-1,6-bisphosphatase to yield fructose 6-phosphate (6C) and releasing P i . Two carbons are then removed from fructose 6-phosphate by transketolase, generating erythrose 4-phosphate (4C); the two carbons are transferred to another molecule of GAP generating xylulose 5-phosphate (5C). Another DHAP molecule, formed from GAP by triose phosphate isomerase is then combined with the erythrose 4-phosphate by aldolase to form sedoheptulose 1,7-bisphosphate (7C). Sedoheptulose 1,7-bisphosphate is then dephosphorylated to sedoheptulose 7-phosphate (7C) by sedoheptulose-1,7-bisphosphatase releasing P i . Sedoheptulose 7-phosphate has two carbons removed by transketolase to produce ribose 5-phosphate (5C) and the two carbons are transferred to another GAP molecule producing another xylulose 5-phosphate (5C). Ribose 5-phosphate and the two molecules of xylulose 5-phosphate (5C) are then converted by phosphopentose isomerase to three molecules of ribulose 5-phosphate (5C). The three ribulose 5-phosphate molecules are then phosphorylated using three ATP by phosphoribulokinase to regenerate three ribulose 1,5-bisphosphate (5C).
Overall the synthesis of 1 mol of GAP requires 9 mol of ATP and 6 mol of NADPH, a required ratio of 1.5 ATP/NADPH. Linear electron transfer is generally thought to supply ATP/NADPH in a ratio of 1.28 (assuming an H + /ATP ratio of 4.67) with the shortfall of ATP believed to be provided by cyclic electron transfer reactions. Since the product of the Calvin cycle is GAP (a 3C sugar) the pathway is often referred to as C 3 photosynthesis and plants that utilize it are called C 3 plants and include many of the world's major crops such as rice, wheat and potato.
Many of the enzymes involved in the Calvin–Benson cycle (e.g. transketolase, glyceraldehyde-3-phosphate dehydrogenase and aldolase) are also involved in the glycolysis pathway of carbohydrate degradation and their activity must therefore be carefully regulated to avoid futile cycling when light is present, i.e. the unwanted degradation of carbohydrate. The regulation of the Calvin–Benson cycle enzymes is achieved by the activity of the light reactions, which modify the environment of the dark reactions (i.e. the stroma). Proton gradient formation across the thylakoid membrane during the light reactions increases the pH and also increases the Mg 2+ concentration in the stroma (as Mg 2+ flows out of the lumen as H + flows in to compensate for the influx of positive charges). In addition, by reducing ferredoxin and NADP + , PSI changes the redox state of the stroma, which is sensed by the regulatory protein thioredoxin. Thioredoxin, pH and Mg 2+ concentration play a key role in regulating the activity of the Calvin–Benson cycle enzymes, ensuring the activity of the light and dark reactions is closely co-ordinated.
It is noteworthy that, despite the complexity of the dark reactions outlined above, the carbon fixation step itself (i.e. the incorporation of CO 2 into carbohydrate) is carried out by a single enzyme, Rubisco. Rubisco is a large multisubunit soluble protein complex found in the chloroplast stroma. The complex consists of eight large (56 kDa) subunits, which contain both catalytic and regulatory domains, and eight small subunits (14 kDa), which enhance the catalytic function of the L subunits ( Figure 17 A). The carboxylation reaction carried out by Rubisco is highly exergonic (Δ G °=−51.9 kJ·mol- 1 ), yet kinetically very slow (just 3 s −1 ) and begins with the protonation of ribulose 1,5-bisphosphate to form an enediolate intermediate which can be combined with CO 2 to form an unstable 6C intermediate that is quickly hydrolysed to yield two 3C 3-phosphoglycerate molecules. The active site in the Rubisco enzyme contains a key lysine residue, which reacts with another (non-substrate) molecule of CO 2 to form a carbamate anion that is then able to bind Mg 2+ . The Mg 2+ in the active site is essential for the catalytic function of Rubisco, playing a key role in binding ribulose 1,5-bisphosphate and activating it such that it readily reacts with CO 2.. Rubisco activity is co-ordinated with that of the light reactions since carbamate formation requires both high Mg 2+ concentration and alkaline conditions, which are provided by the light-driven changes in the stromal environment discussed above ( Figure 17 B).

( A ) Structure of the Rubisco enzyme (the large subunits are shown in blue and the small subunits in green); four of each type of subunit are visible in the image. Drawn from PDB code 1RXO. ( B ) Activation of the lysine residue within the active site of Rubisco occurs via elevated stromal pH and Mg 2+ concentration as a result of the activity of the light reactions.
In addition to carboxylation, Rubisco also catalyses a competitive oxygenation reaction, known as photorespiration, that results in the combination of ribulose 1,5-bisphosphate with O 2 rather than CO 2 . In the oxygenation reaction, one rather than two molecules of 3-phosphoglycerate and one molecule of a 2C sugar known as phosphoglycolate are produced by Rubisco. The phosphoglycolate must be converted in a series of reactions that regenerate one molecule of 3-phosphoglycerate and one molecule of CO 2 . These reactions consume additional ATP and thus result in an energy loss to the plant. Although the oxygenation reaction of Rubisco is much less favourable than the carboxylation reaction, the relatively high concentration of O 2 in the leaf (250 μM) compared with CO 2 (10 μM) means that a significant amount of photorespiration is always occurring. Under normal conditions, the ratio of carboxylation to oxygenation is between 3:1 and 4:1. However, this ratio can be decreased with increasing temperature due to decreased CO 2 concentration in the leaf, a decrease in the affinity of Rubisco for CO 2 compared with O 2 and an increase in the maximum rate of the oxygenation reaction compared with the carboxylation reaction. The inefficiencies of the Rubisco enzyme mean that plants must produce it in very large amounts (∼30–50% of total soluble protein in a spinach leaf) to achieve the maximal photosynthetic rate.
CO 2 -concentrating mechanisms
To counter photorespiration, plants, algae and cyanobacteria have evolved different CO 2 -concentrating mechanisms CCMs that aim to increase the concentration of CO 2 relative to O 2 in the vicinity of Rubisco. One such CCM is C 4 photosynthesis that is found in plants such as maize, sugar cane and savanna grasses. C 4 plants show a specialized leaf anatomy: Kranz anatomy ( Figure 18 ). Kranz, German for wreath, refers to a bundle sheath of cells that surrounds the central vein within the leaf, which in turn are surrounded by the mesophyll cells. The mesophyll cells in such leaves are rich in the enzyme phosphoenolpyruvate (PEP) carboxylase, which fixes CO 2 into a 4C carboxylic acid: oxaloaceatate. The oxaloacetate formed by the mesophyll cells is reduced using NADPH to malate, another 4C acid: malate. The malate is then exported from the mesophyll cells to the bundle sheath cells, where it is decarboxylated to pyruvate thus regenerating NADPH and CO 2 . The CO 2 is then utilized by Rubisco in the Calvin cycle. The pyruvate is in turn returned to the mesophyll cells where it is phosphorylated using ATP to reform PEP ( Figure 19 ). The advantage of C 4 photosynthesis is that CO 2 accumulates at a very high concentration in the bundle sheath cells that is then sufficient to allow Rubisco to operate efficiently.
Diagram of a C 4 plant leaf showing Kranz anatomy

The C 4 pathway (NADP + –malic enzyme type) for fixation of CO 2

Plants growing in hot, bright and dry conditions inevitably have to have their stomata closed for large parts of the day to avoid excessive water loss and wilting. The net result is that the internal CO 2 concentration in the leaf is very low, meaning that C 3 photosynthesis is not possible. To counter this limitation, another CCM is found in succulent plants such as cacti. The Crassulaceae fix CO 2 into malate during the day via PEP carboxylase, store it within the vacuole of the plant cell at night and then release it within their tissues by day to be fixed via normal C 3 photosynthesis. This is termed crassulacean acid metabolism (CAM).
This article is a reviewed, revised and updated version of the following ‘Biochemistry Across the School Curriculum’ (BASC) booklet: Weaire, P.J. (1994) Photosynthesis . For further information and to provide feedback on this or any other Biochemical Society education resource, please contact [email protected]. For further information on other Biochemical Society publications, please visit www.biochemistry.org/publications .
adenosine diphosphate
adenosine triphosphate
carbohydrate
cytochrome b 6 f
dihydroxyacetone phosphate
excitation energy transfer
ferredoxin–NADP + reductase
glyceraldehyde 3-phosphate
light-harvesting complex
nicotinomide–adenine dinucleotide phosphate
phosphoenolpyruvate
inorganic phosphate
reaction centre
ribulose-1,5-bisphosphate carboxylase/oxygenase
I thank Professor Colin Osborne (University of Sheffield, Sheffield, U.K.) for useful discussions on the article, Dr Dan Canniffe (Penn State University, Pennsylvania, PA, U.S.A.) for providing pure pigment spectra and Dr P.J. Weaire (Kingston University, Kingston-upon-Thames, U.K.) for his original Photosynthesis BASC article (1994) on which this essay is partly based.
The Author declares that there are no competing interests associated with this article.
Get Email Alerts
- Online ISSN 1744-1358
- Print ISSN 0071-1365
- Submit Your Work
- Language-editing services
- Recommend to Your Librarian
- Request a free trial
- Accessibility
- Sign up for alerts
- Sign up to our mailing list
- The Biochemist Blog
- Biochemical Society Membership
- Publishing Life Cycle
- Biochemical Society Events
- About Portland Press
- Portland Press Tel
- +44 (0)20 3880 2795
- Portland Press Company no. 02453983
- Biochemical Society Tel
- +44 (0)20 3880 2793
- Email: [email protected]
- Biochemical Society Company no. 00892796
- Registered Charity no. 253894
- VAT no. GB 523 2392 69
- Privacy and cookies
- © Copyright 2024 Portland Press
This Feature Is Available To Subscribers Only
Sign In or Create an Account
If you're seeing this message, it means we're having trouble loading external resources on our website.
If you're behind a web filter, please make sure that the domains *.kastatic.org and *.kasandbox.org are unblocked.
To log in and use all the features of Khan Academy, please enable JavaScript in your browser.
Middle school biology - NGSS
Course: middle school biology - ngss > unit 5, photosynthesis in ecosystems.
- Understand: photosynthesis in ecosystems
Key points:
- Energy enters an ecosystem when light energy from the sun is transformed into chemical energy. This happens during photosynthesis .
- Photosynthesis is carried out by photosynthetic organisms .
- Photosynthetic organisms take in and use carbon dioxide and water from the air and soil.
- Photosynthetic organisms release oxygen into the air. Organisms throughout the ecosystem use this oxygen to breathe.
- Photosynthetic organisms produce sugars, which become part of the organism’s biomass . When the photosynthetic organism is eaten, its biomass provides matter and energy to the organism that eats it.
Want to join the conversation?
- Upvote Button navigates to signup page
- Downvote Button navigates to signup page
- Flag Button navigates to signup page

Understanding Global Change
Discover why the climate and environment changes, your place in the Earth system, and paths to a resilient future.
Photosynthesis

Photosynthesis is the processes of using sunlight to convert chemical compounds (specifically carbon dioxide and water ) into food . Photosynthesizing organisms (plants, algae, and bacteria) provide most of the chemical energy that flows through the biosphere. They also produced most of the biomass that led to the fossil fuels that power much of our modern world. Photosynthesis takes place on land, in the ocean, and in freshwater environments. The first photosynthesizing single-celled bacteria evolved over 3.5 billion years ago. The subsequent rise in atmospheric oxygen (a byproduct of photosynthesis) about a billion years later played a major role in shaping the evolution of life on Earth over the last 2.5 billion years. Today the vast majority of land, freshwater, and oceanic organisms require oxygen for respiration , the biochemical process that generates energy from food.
On this page:
What is photosynthesis, earth system model about photosynthesis, explore the earth system, investigate, links to learn more.
For the classroom:
- Teaching Resources

Global Change Infographic
Photosynthesus is an essential part of How the Earth System Works. Click the image on the left to open the Understanding Global Change Infographic . Locate the photosynthesis icon and identify other Earth system processes and phenomena that cause changes to, or are affected by, photosynthesis.
Photosynthesis is the chemical process by which plants, algae, and some bacteria use the energy from sunlight to transform carbon dioxide (a greenhouse gas ) from the atmosphere, and water , into organic compounds such as sugars. These sugars are then used to make complex carbohydrates, lipids, and proteins, as well as the wood, leaves, and roots of plants. The amount of organic matter made by photosynthesizing organisms in an ecosystem is defined as the productivity of that ecosystem. Energy flows through the biosphere as organisms (including some animals) eat photosynthesizing organisms (called herbivores), and as organisms then eat those herbivores (carnivores) , etc., to get their energy for growth, reproduction, and other functions. This energy is acquired through the process of cellular respiration , which usually requires oxygen. Oxygen is a byproduct of photosynthesis. About 70% of the oxygen in the atmosphere that we breathe comes from algae in the ocean. Atmospheric oxygen from photosynthesis also forms the ozone layer , which protects organisms from harmful high-energy ultraviolet (UV) radiation from the Sun . Because photosynthesis also requires water , the availability of water affects the productivity and biomass of the ecosystem, which in turn affects how much and how rapidly water cycles through the ecosystem.
Fossil fuels are derived from the burial of photosynthetic organisms, including plants on land (which primarily form coal) and plankton in the oceans (which primarily form oil and natural gas). While buried, the carbon in the organic material is removed from the carbon cycle for thousands of years to hundreds of millions of years. The burning of fossil fuels has dramatically increased the exchange of carbon from the ground back into the atmosphere and oceans. This return of carbon back into atmosphere as carbon dioxide is occurring at a rate that is hundreds to thousands of times faster than it took to bury it, and much faster than it can be removed by photosynthesis or weathering . Thus, the carbon dioxide released from the burning of fossil fuels is accumulating in the atmosphere, increasing average temperatures and causing ocean acidification .

A simplified diagram showing the overall inputs – carbon dioxide, water, and sunlight, and products – oxygen and sugar (glucose), of photosynthesis.
The rate of photosynthesis in ecosystems is affected by various environmental conditions, including:
- Climatic conditions, such as the amount of sunlight available at different latitudes , temperature , and precipitation For example, ecosystems at low latitudes, such as tropical rainforests, have higher productivity and biomass than ecosystems near the poles because of they receive more sunlight and rainfall than regions at higher latitudes.
- Nutrients , especially nitrogen and phosphorus , which when limited can decrease productivity, but when abundant can increase productivity and biomass. Photosynthesizing organisms extract nutrients from the environment, and return them to the soil when they die and decay.
- Numerous other abiotic environmental factors, including soil quality (often related to nutrient levels), wildfires , water acidity , and oxygen levels .
- Species interactions , including the resources species provide for each other, and how they compete for resources such as water, light, and/or space. Species that reduce or increase the success of other species alter population sizes , thus affecting productivity and biomass .
- Evolutionary processes that can change the growth and reproduction rates of photosynthesizing organisms over time, as well as the growth and reproduction of rates of the organisms that eat them.
Humans have altered the rate of photosynthesis, and in turn productivity , in ecosystems through a variety of activities, including:
- Deforestation , habitat destruction , and urbanization , which remove plants and trees from the environment and disrupt ecosystems.
- Agricultural activities that increase the amount of crops available to feed the growing global human population .
- The use of fertilizers for agricultural activities that increase the amount of nutrients , especially nitrogen and phosphorous , in soil or water. These nutrients increase plant and algae growth, including growth of species that are toxic to other organisms. Increased nutrients is not always a good thing. For example, in aquatic environments, nutrient-rich runoff can cause large amounts of algae to grow – when these algae die, they are consumed by bacteria which can reduce oxygen levels in the water, killing fish and other species. This process is known as eutrophication.
- Human freshwater use , which can limit the amount of water available for plants and trees in an ecosystem.
- The release of pollutants and waste , which can reduce growth and reproduction or kill plants.
- Activities that release carbon dioxide and other greenhouse gases that cause global warming, such as the burning of fossil fuels , agricultural activities , and deforestation . Increasing carbon dioxide levels may increase photosynthesis rates in some plants, but this can also make plants less nutritious . Increasing average global land and ocean temperatures and changes in precipitation patterns also affect plant and algae growth, and can make certain species more susceptible to disease .
- Activities such as the burning of fossil fuels , agricultural activities , and deforestation that release carbon dioxide into the atmosphere, which is absorbed by the ocean causing acidification . The decreasing pH of ocean waters (along with ocean warming) causes physiological stress for many plant and algae species, which can decrease growth, reproduction, species population sizes, and biomass .
- The increase in carbon dioxide to the atmosphere is also thought to impact global photosynthesis rates. During photosynthesis, plants convert carbon dioxide to biomass such as sugars and wood. However, the same enzyme (rubisco) that fixes carbon dioxide can also use oxygen. When oxygen is used, plants undergo a process known as photorespiration where biomass is not produced and instead carbon dioxide is emitted to the atmosphere (see this teaching resource for more information). Photorespiration is often considered a negative process for plants. It has been proposed that as carbon dioxide levels rise in the atmosphere rates of photorespiration will decrease and rates of photosynthesis will increase. This change is termed carbon dioxide fertilization and demonstrates the complex interactions between life and climate change.
- Introducing invasive species that compete with native plant or algae species for nutrients, water, light, or other resources, reducing native species populations.
The Earth system model below includes some of the processes and phenomena related to photosynthesis. These processes operate at various rates and on different spatial and temporal scales. For example, carbon dioxide is transferred among plants and animals over relatively short time periods (hours-weeks), but the deforestation alters ecosystems over decades to centuries, or longer. Can you think of additional cause and effect relationships between photosynthesis and other processes in the Earth system?
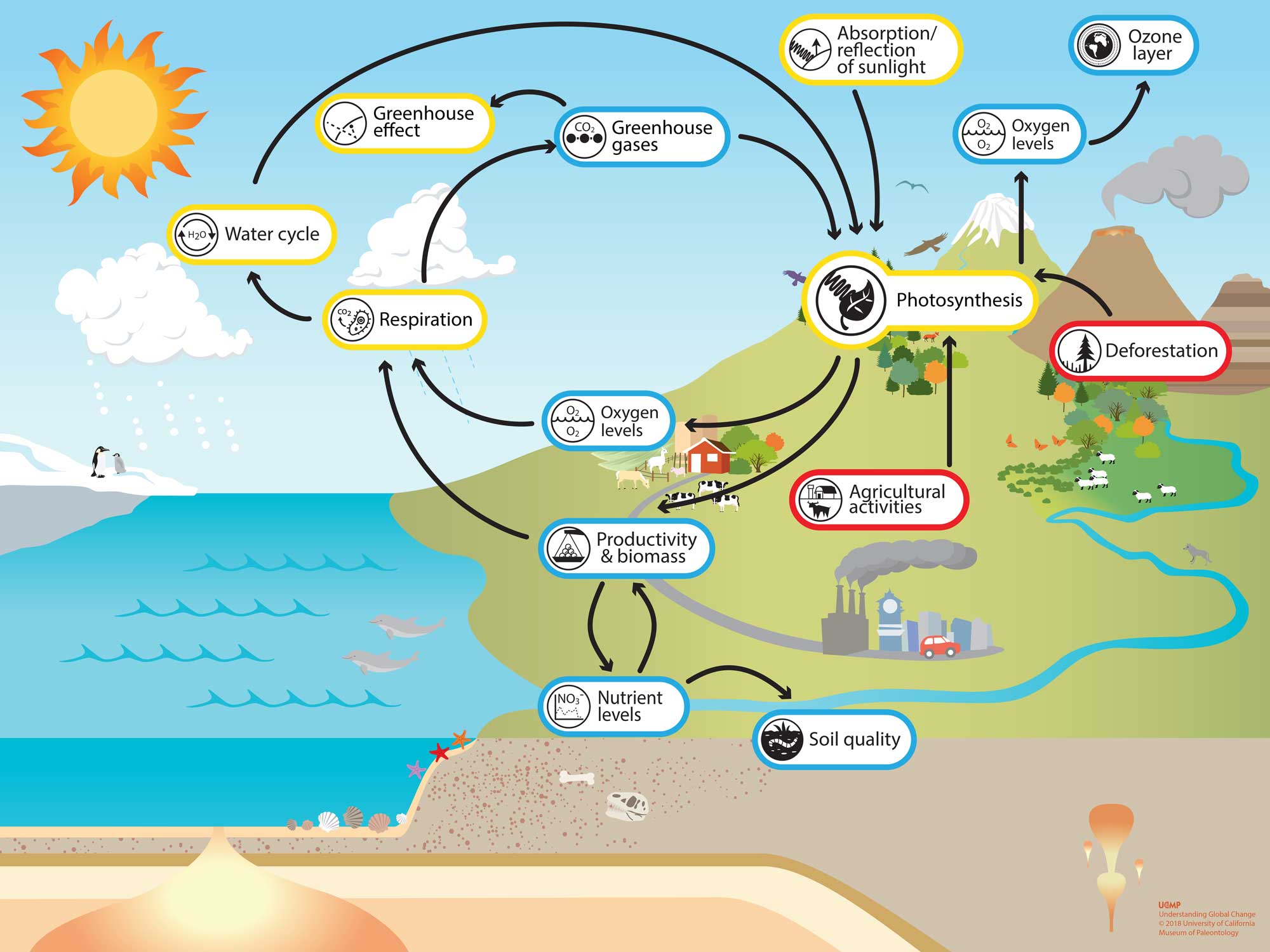
Click the bolded terms (e.g. respiration , productivity and biomass , and burning of fossil fuels ) on this page to learn more about these process and phenomena. Alternatively, explore the Understanding Global Change Infographic and find new topics that are of interest and/or locally relevant to you.
Learn more in these real-world examples, and challenge yourself to construct a model that explains the Earth system relationships.
- The bacteria that changed the world
- New York Times: Antarctic Ice Reveals Earth’s Accelerating Plant Growth
- USGCRP: Climate and Health Assessment, Food Safety, Nutrition, and Distribution
- HHMI BioInteractive: Photosynthesis
- Evolution Connection: More on photorespiration
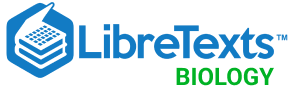
- school Campus Bookshelves
- menu_book Bookshelves
- perm_media Learning Objects
- login Login
- how_to_reg Request Instructor Account
- hub Instructor Commons
Margin Size
- Download Page (PDF)
- Download Full Book (PDF)
- Periodic Table
- Physics Constants
- Scientific Calculator
- Reference & Cite
- Tools expand_more
- Readability
selected template will load here
This action is not available.
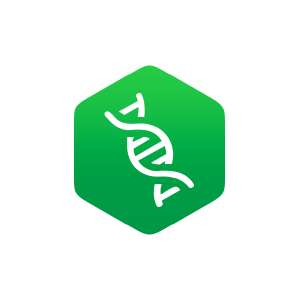
8.1: Introduction to Photosynthesis
- Last updated
- Save as PDF
- Page ID 75475
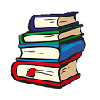
\( \newcommand{\vecs}[1]{\overset { \scriptstyle \rightharpoonup} {\mathbf{#1}} } \)
\( \newcommand{\vecd}[1]{\overset{-\!-\!\rightharpoonup}{\vphantom{a}\smash {#1}}} \)
\( \newcommand{\id}{\mathrm{id}}\) \( \newcommand{\Span}{\mathrm{span}}\)
( \newcommand{\kernel}{\mathrm{null}\,}\) \( \newcommand{\range}{\mathrm{range}\,}\)
\( \newcommand{\RealPart}{\mathrm{Re}}\) \( \newcommand{\ImaginaryPart}{\mathrm{Im}}\)
\( \newcommand{\Argument}{\mathrm{Arg}}\) \( \newcommand{\norm}[1]{\| #1 \|}\)
\( \newcommand{\inner}[2]{\langle #1, #2 \rangle}\)
\( \newcommand{\Span}{\mathrm{span}}\)
\( \newcommand{\id}{\mathrm{id}}\)
\( \newcommand{\kernel}{\mathrm{null}\,}\)
\( \newcommand{\range}{\mathrm{range}\,}\)
\( \newcommand{\RealPart}{\mathrm{Re}}\)
\( \newcommand{\ImaginaryPart}{\mathrm{Im}}\)
\( \newcommand{\Argument}{\mathrm{Arg}}\)
\( \newcommand{\norm}[1]{\| #1 \|}\)
\( \newcommand{\Span}{\mathrm{span}}\) \( \newcommand{\AA}{\unicode[.8,0]{x212B}}\)
\( \newcommand{\vectorA}[1]{\vec{#1}} % arrow\)
\( \newcommand{\vectorAt}[1]{\vec{\text{#1}}} % arrow\)
\( \newcommand{\vectorB}[1]{\overset { \scriptstyle \rightharpoonup} {\mathbf{#1}} } \)
\( \newcommand{\vectorC}[1]{\textbf{#1}} \)
\( \newcommand{\vectorD}[1]{\overrightarrow{#1}} \)
\( \newcommand{\vectorDt}[1]{\overrightarrow{\text{#1}}} \)
\( \newcommand{\vectE}[1]{\overset{-\!-\!\rightharpoonup}{\vphantom{a}\smash{\mathbf {#1}}}} \)

The processes in all organisms—from bacteria to humans—require energy. To get this energy, many organisms access stored energy by eating, that is, by ingesting other organisms. But where does the stored energy in food originate? All of this energy can be traced back to photosynthesis.
Contributors and Attributions
Connie Rye (East Mississippi Community College), Robert Wise (University of Wisconsin, Oshkosh), Vladimir Jurukovski (Suffolk County Community College), Jean DeSaix (University of North Carolina at Chapel Hill), Jung Choi (Georgia Institute of Technology), Yael Avissar (Rhode Island College) among other contributing authors. Original content by OpenStax (CC BY 4.0; Download for free at http://cnx.org/contents/[email protected] ).
- Biology Article
Photosynthesis
Photosynthesis is a process by which phototrophs convert light energy into chemical energy, which is later used to fuel cellular activities. The chemical energy is stored in the form of sugars, which are created from water and carbon dioxide.
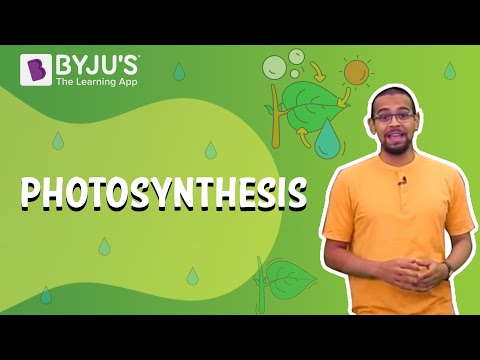
Table of Contents
- What is Photosynthesis?
- Site of photosynthesis
Photosynthesis definition states that the process exclusively takes place in the chloroplasts through photosynthetic pigments such as chlorophyll a, chlorophyll b, carotene and xanthophyll. All green plants and a few other autotrophic organisms utilize photosynthesis to synthesize nutrients by using carbon dioxide, water and sunlight. The by-product of the photosynthesis process is oxygen.Let us have a detailed look at the process, reaction and importance of photosynthesis.
What Is Photosynthesis in Biology?
The word “ photosynthesis ” is derived from the Greek words phōs (pronounced: “fos”) and σύνθεσις (pronounced: “synthesis “) Phōs means “light” and σύνθεσις means, “combining together.” This means “ combining together with the help of light .”
Photosynthesis also applies to other organisms besides green plants. These include several prokaryotes such as cyanobacteria, purple bacteria and green sulfur bacteria. These organisms exhibit photosynthesis just like green plants.The glucose produced during photosynthesis is then used to fuel various cellular activities. The by-product of this physio-chemical process is oxygen.

A visual representation of the photosynthesis reaction
- Photosynthesis is also used by algae to convert solar energy into chemical energy. Oxygen is liberated as a by-product and light is considered as a major factor to complete the process of photosynthesis.
- Photosynthesis occurs when plants use light energy to convert carbon dioxide and water into glucose and oxygen. Leaves contain microscopic cellular organelles known as chloroplasts.
- Each chloroplast contains a green-coloured pigment called chlorophyll. Light energy is absorbed by chlorophyll molecules whereas carbon dioxide and oxygen enter through the tiny pores of stomata located in the epidermis of leaves.
- Another by-product of photosynthesis is sugars such as glucose and fructose.
- These sugars are then sent to the roots, stems, leaves, fruits, flowers and seeds. In other words, these sugars are used by the plants as an energy source, which helps them to grow. These sugar molecules then combine with each other to form more complex carbohydrates like cellulose and starch. The cellulose is considered as the structural material that is used in plant cell walls.
Where Does This Process Occur?
Chloroplasts are the sites of photosynthesis in plants and blue-green algae. All green parts of a plant, including the green stems, green leaves, and sepals – floral parts comprise of chloroplasts – green colour plastids. These cell organelles are present only in plant cells and are located within the mesophyll cells of leaves.
Also Read: Photosynthesis Early Experiments
Photosynthesis Equation
Photosynthesis reaction involves two reactants, carbon dioxide and water. These two reactants yield two products, namely, oxygen and glucose. Hence, the photosynthesis reaction is considered to be an endothermic reaction. Following is the photosynthesis formula:
Unlike plants, certain bacteria that perform photosynthesis do not produce oxygen as the by-product of photosynthesis. Such bacteria are called anoxygenic photosynthetic bacteria. The bacteria that do produce oxygen as a by-product of photosynthesis are called oxygenic photosynthetic bacteria.
Structure Of Chlorophyll
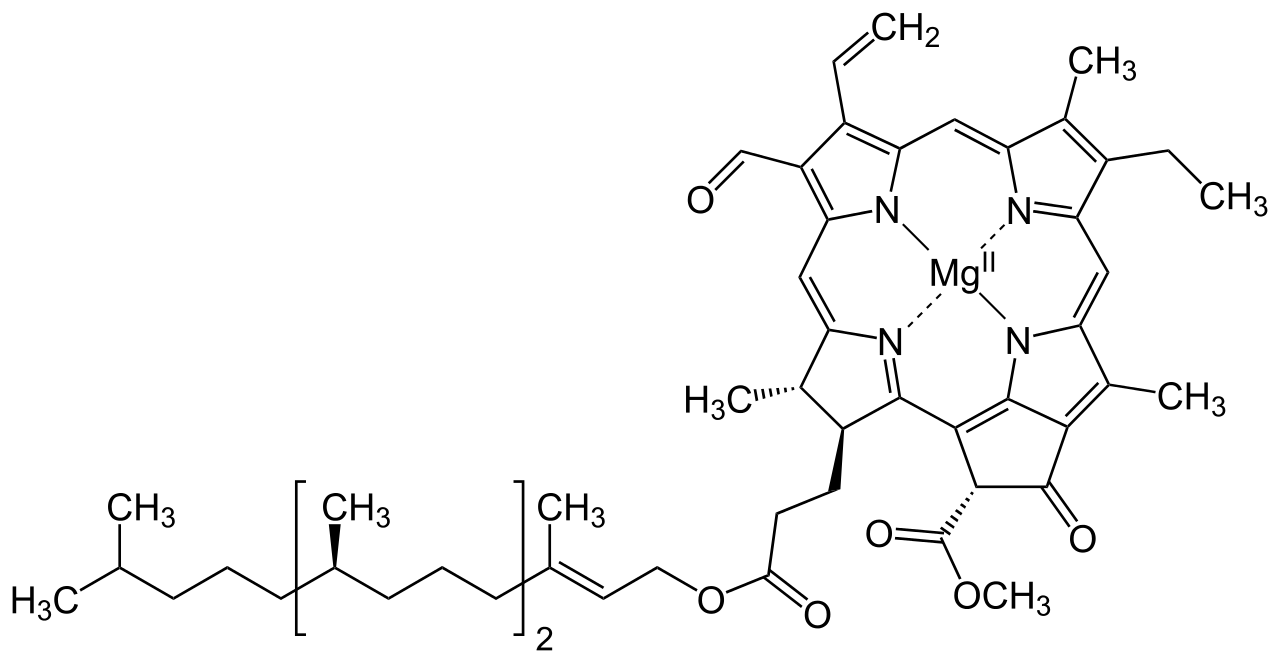
The structure of Chlorophyll consists of 4 nitrogen atoms that surround a magnesium atom. A hydrocarbon tail is also present. Pictured above is chlorophyll- f, which is more effective in near-infrared light than chlorophyll- a
Chlorophyll is a green pigment found in the chloroplasts of the plant cell and in the mesosomes of cyanobacteria. This green colour pigment plays a vital role in the process of photosynthesis by permitting plants to absorb energy from sunlight. Chlorophyll is a mixture of chlorophyll- a and chlorophyll- b .Besides green plants, other organisms that perform photosynthesis contain various other forms of chlorophyll such as chlorophyll- c1 , chlorophyll- c2 , chlorophyll- d and chlorophyll- f .
Also Read: Biological Pigments
Process Of Photosynthesis
At the cellular level, the photosynthesis process takes place in cell organelles called chloroplasts. These organelles contain a green-coloured pigment called chlorophyll, which is responsible for the characteristic green colouration of the leaves.
As already stated, photosynthesis occurs in the leaves and the specialized cell organelles responsible for this process is called the chloroplast. Structurally, a leaf comprises a petiole, epidermis and a lamina. The lamina is used for absorption of sunlight and carbon dioxide during photosynthesis.
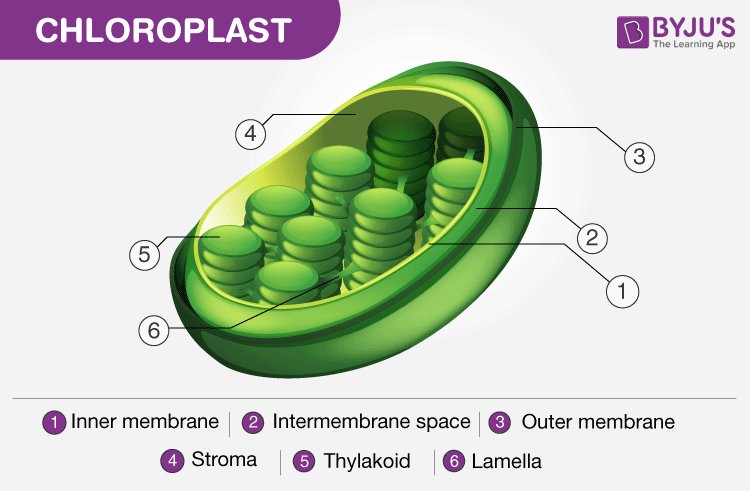
Structure of Chloroplast. Note the presence of the thylakoid
“Photosynthesis Steps:”
- During the process of photosynthesis, carbon dioxide enters through the stomata, water is absorbed by the root hairs from the soil and is carried to the leaves through the xylem vessels. Chlorophyll absorbs the light energy from the sun to split water molecules into hydrogen and oxygen.
- The hydrogen from water molecules and carbon dioxide absorbed from the air are used in the production of glucose. Furthermore, oxygen is liberated out into the atmosphere through the leaves as a waste product.
- Glucose is a source of food for plants that provide energy for growth and development , while the rest is stored in the roots, leaves and fruits, for their later use.
- Pigments are other fundamental cellular components of photosynthesis. They are the molecules that impart colour and they absorb light at some specific wavelength and reflect back the unabsorbed light. All green plants mainly contain chlorophyll a, chlorophyll b and carotenoids which are present in the thylakoids of chloroplasts. It is primarily used to capture light energy. Chlorophyll-a is the main pigment.
The process of photosynthesis occurs in two stages:
- Light-dependent reaction or light reaction
- Light independent reaction or dark reaction
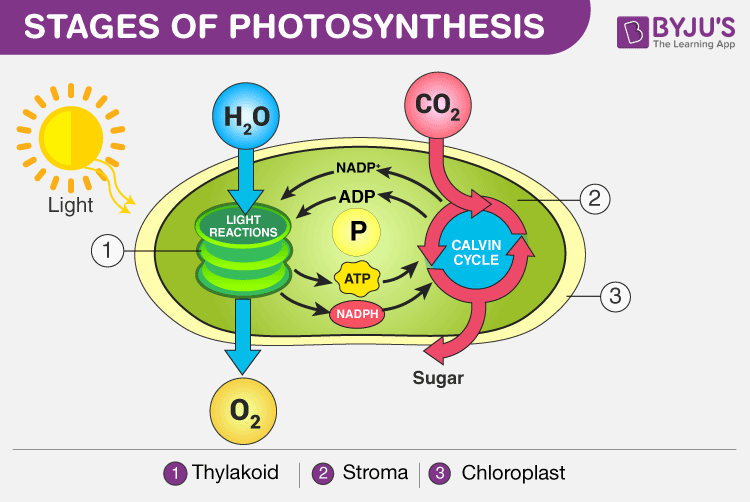
Stages of Photosynthesis in Plants depicting the two phases – Light reaction and Dark reaction
Light Reaction of Photosynthesis (or) Light-dependent Reaction
- Photosynthesis begins with the light reaction which is carried out only during the day in the presence of sunlight. In plants, the light-dependent reaction takes place in the thylakoid membranes of chloroplasts.
- The Grana, membrane-bound sacs like structures present inside the thylakoid functions by gathering light and is called photosystems.
- These photosystems have large complexes of pigment and proteins molecules present within the plant cells, which play the primary role during the process of light reactions of photosynthesis.
- There are two types of photosystems: photosystem I and photosystem II.
- Under the light-dependent reactions, the light energy is converted to ATP and NADPH, which are used in the second phase of photosynthesis.
- During the light reactions, ATP and NADPH are generated by two electron-transport chains, water is used and oxygen is produced.
The chemical equation in the light reaction of photosynthesis can be reduced to:
2H 2 O + 2NADP+ + 3ADP + 3Pi → O 2 + 2NADPH + 3ATP
Dark Reaction of Photosynthesis (or) Light-independent Reaction
- Dark reaction is also called carbon-fixing reaction.
- It is a light-independent process in which sugar molecules are formed from the water and carbon dioxide molecules.
- The dark reaction occurs in the stroma of the chloroplast where they utilize the NADPH and ATP products of the light reaction.
- Plants capture the carbon dioxide from the atmosphere through stomata and proceed to the Calvin photosynthesis cycle.
- In the Calvin cycle , the ATP and NADPH formed during light reaction drive the reaction and convert 6 molecules of carbon dioxide into one sugar molecule or glucose.
The chemical equation for the dark reaction can be reduced to:
3CO 2 + 6 NADPH + 5H 2 O + 9ATP → G3P + 2H+ + 6 NADP+ + 9 ADP + 8 Pi
* G3P – glyceraldehyde-3-phosphate
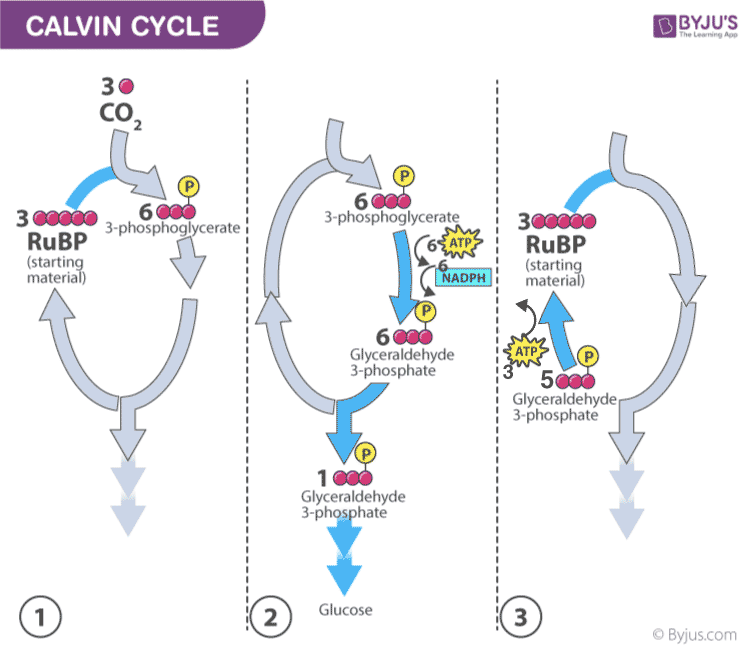
Calvin photosynthesis Cycle (Dark Reaction)
Also Read: Cyclic And Non-Cyclic Photophosphorylation
Importance of Photosynthesis
- Photosynthesis is essential for the existence of all life on earth. It serves a crucial role in the food chain – the plants create their food using this process, thereby, forming the primary producers.
- Photosynthesis is also responsible for the production of oxygen – which is needed by most organisms for their survival.
Frequently Asked Questions
1. what is photosynthesis explain the process of photosynthesis., 2. what is the significance of photosynthesis, 3. list out the factors influencing photosynthesis., 4. what are the different stages of photosynthesis, 5. what is the calvin cycle, 6. write down the photosynthesis equation..
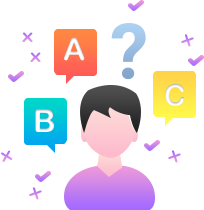
Put your understanding of this concept to test by answering a few MCQs. Click ‘Start Quiz’ to begin!
Select the correct answer and click on the “Finish” button Check your score and answers at the end of the quiz
Visit BYJU’S for all Biology related queries and study materials
Your result is as below
Request OTP on Voice Call
Leave a Comment Cancel reply
Your Mobile number and Email id will not be published. Required fields are marked *
Post My Comment

very useful
It’s very helpful ☺️
Please What Is Meant By 300-400 PPM
PPM stands for Parts-Per-Million. It corresponds to saying that 300 PPM of carbon dioxide indicates that if one million gas molecules are counted, 300 out of them would be carbon dioxide. The remaining nine hundred ninety-nine thousand seven hundred are other gas molecules.
Thank you very much Byju’s! I couldn’t find the answer anywhere. But luckily I hit upon this website. Awesome explanation and illustration.
byjus = Wow!
It helps me a lot thank you
Thanks in a million I love Byjus!
Super Byjus
Thanks helped a lot
Very interesting and helpful site.
Nice it is very uesful
It’s very useful 👍 Thank you Byju’s
Thank you very much Byju’s! I couldn’t find the answer anywhere. But luckily I hit upon this website. Awesome explanation and illustration.
Thank you BYJU’S for helping me in further clarifying my concepts
Excellent material easy to understand
Indeed, it’s precise and understandable. I like it.

- Share Share
Register with BYJU'S & Download Free PDFs
Register with byju's & watch live videos.


Essay on Photosynthesis
Students are often asked to write an essay on Photosynthesis in their schools and colleges. And if you’re also looking for the same, we have created 100-word, 250-word, and 500-word essays on the topic.
Let’s take a look…
100 Words Essay on Photosynthesis
What is photosynthesis.
Photosynthesis is how plants make their own food using sunlight. It happens in the leaves of plants. Tiny parts inside the leaves, called chloroplasts, use sunlight to turn water and carbon dioxide from the air into sugar and oxygen. The sugar is food for the plant.
The Ingredients
The main things needed for photosynthesis are sunlight, water, and carbon dioxide. Roots soak up water from the soil. Leaves take in carbon dioxide from the air. Then, using sunlight, plants create food and release oxygen.
The Process
In the chloroplasts, sunlight energy is changed into chemical energy. This energy turns water and carbon dioxide into glucose, a type of sugar. Oxygen is made too, which goes into the air for us to breathe.
Why It’s Important
Photosynthesis is vital for life on Earth. It gives us food and oxygen. Without it, there would be no plants, and without plants, animals and people would not survive. It also helps take in carbon dioxide, which is good for the Earth.
250 Words Essay on Photosynthesis
Photosynthesis is a process used by plants, algae, and some bacteria to turn sunlight, water, and carbon dioxide into food and oxygen. Think of it like a recipe that plants use to make their own food. This happens in the leaves of plants, which have a green substance called chlorophyll.
Why is Photosynthesis Important?
This process is very important because it is the main way plants make food for themselves and for us, too. Without photosynthesis, plants could not grow, and without plants, animals and humans would not have oxygen to breathe or food to eat.
How Photosynthesis Works
Photosynthesis happens in two main stages. In the first stage, the plant captures sunlight with its leaves. The sunlight gives the plant energy to split water inside its leaves into hydrogen and oxygen. The oxygen is released into the air, and the hydrogen is used in the next stage.
In the second stage, the plant mixes the hydrogen with carbon dioxide from the air to make glucose, which is a type of sugar that plants use for energy. This energy helps the plant to grow, make flowers, and produce seeds.
The Cycle of Life
Photosynthesis is a key part of the cycle of life on Earth. By making food and oxygen, plants support life for all creatures. When animals eat plants, they get the energy from the plants, and when animals breathe, they use the oxygen that plants release. It’s a beautiful cycle that keeps the planet alive.

500 Words Essay on Photosynthesis
Photosynthesis is a process used by plants, algae, and some bacteria to turn sunlight, water, and carbon dioxide into food and oxygen. This happens in the green parts of plants, mainly the leaves. The green color comes from chlorophyll, a special substance in the leaves that captures sunlight.
The Ingredients of Photosynthesis
To make their food, plants need three main things: sunlight, water, and carbon dioxide. Sunlight is the energy plants use to create their food. They get water from the ground through their roots. Carbon dioxide, a gas found in the air, is taken in through tiny holes in the leaves called stomata.
The Photosynthesis Recipe
When sunlight hits the leaves, the chlorophyll captures it and starts the food-making process. The energy from the sunlight turns water and carbon dioxide into glucose, a type of sugar that plants use for energy, and oxygen, which is released into the air. This process is like a recipe that plants follow to make their own food.
The Importance of Photosynthesis
Photosynthesis is very important for life on Earth. It gives us oxygen, which we need to breathe. Plants use the glucose they make for growth and to build other important substances like cellulose, which they use to make their cell walls. Without photosynthesis, there would be no food for animals or people, and no oxygen to breathe.
The Benefits to the Environment
Photosynthesis also helps the environment. Plants take in carbon dioxide, which is a gas that can make the Earth warmer when there is too much of it in the air. By using carbon dioxide to make food, plants help keep the air clean and the Earth’s temperature just right.
Photosynthesis and the Food Chain
All living things need energy to survive, and this energy usually comes from food. Plants are at the bottom of the food chain because they can make their own food using photosynthesis. Animals that eat plants get energy from the glucose in the plants. Then, animals that eat other animals get this energy too. So, photosynthesis is the start of the food chain that feeds almost every living thing on Earth.
Photosynthesis in Our Lives
Photosynthesis affects our lives in many ways. It gives us fruits, vegetables, and grains to eat. Trees and plants also give us wood, paper, and other materials. Plus, they provide shade and help make the air fresh and clean.
In conclusion, photosynthesis is a vital process that allows plants to make food and oxygen using sunlight, water, and carbon dioxide. It is the foundation of the food chain and has a big impact on the environment and our lives. Understanding photosynthesis helps us appreciate how important plants are and why we need to take care of them and the environment they live in.
That’s it! I hope the essay helped you.
If you’re looking for more, here are essays on other interesting topics:
- Essay on Gender Equality And Women’s Empowerment
- Essay on Gender Equality And Sustainable Development
- Essay on Exciting Cricket Match
Apart from these, you can look at all the essays by clicking here .
Happy studying!
Leave a Reply Cancel reply
Your email address will not be published. Required fields are marked *
Save my name, email, and website in this browser for the next time I comment.

- BiologyDiscussion.com
- Follow Us On:
- Google Plus
- Publish Now
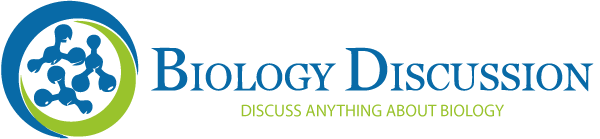
Photosynthesis: Essay on Photosynthesis (2098 Words)
ADVERTISEMENTS:
Here is your essay on Photosynthesis!
[I] Photosynthesis:
Photosynthesis is one of the most fundamental biological reactions.
The chlorophyll bearing plants trap the free energy of sunlight as photons and transform and store it as chemical potential energy by combining CO 2 and water.
The end products of photosynthesis are carbohydrates with loss of oxygen. These directly or indirectly serve as the source of energy for all living beings, except chemosynthetic bacteria.

Image Courtesy : co2crc.com.au/Photosynthesis_media.jpg
[II] Food storage :
Some unpigmented plastids like leucoplasts store the essential food materials like protein, oil and starch. Later on these are used during germination of seeds and development.
[III] Hereditary carrier :
Recent studies show that these plastids, like chromosomes, are transmitted directly to the daughter cells during cell division. Cytoplasmic inheritance of plastids in Mirabilis is the well-known example. They produce phenotypic effects in Oenothera and other plants.
[IV] Chloroplasts as semiautonomous organoid :
The chloroplast matrix contains dissolved salts and enzymes of photosynthesis. Besides these, like mitochondria, it contains RNA, DNA and ribosomes, and is capable of carrying on protein synthesis.
The chloroplast ribosomes are of the same size as ribosomes in prokaryotes. Chloroplasts are also semi-autonomous like mitochondria. They can grow and divide, and their DNA contains a portion of the genetic information needed for the synthesis of chloroplast proteins.
[V] Inheritance of chloroplasts :
Cells have the capacity to outgrow their chloroplasts and the rate of multiplication of chloroplasts is partly independent of the rate of multiplication of entire cells. Brawerman and Chargaff (1960) discovered it in Euglena gracilis after a temperature shock.
Cells which were permitted to multiply rapidly became irreversibly bleached, whereas cells prevented from dividing regained their normal ability to produce chloroplasts. They concluded that Euglena contains an autonomously replicating factor which is necessary for chloroplast formation.
[VI] DNA in chloroplasts :
Chloroplasts contain both DNA and the necessary mechanism for synthesizing specific RNA’s and proteins from a DNA template. DNA is found in chloroplasts (Stocking and Gifford, 1959). Ris and Plaut (1962) have also found DNA in the chloroplasts of alga Chlamydomonas. It has now been generally accepted that characteristic chloroplast DNA’s or chloroplast chromosomes occur in the photosynthetic organelles of algae and higher plants.
According to Brawerman (1966) this DNA differs from nuclear DNA in GC (guanosine and cytosine) content. Chloroplasts also contain a DNA-dependent RNA polymerase; it appears that specific RNA’s are synthesized from chloroplast DNA as a template (Kirk 1966). Chloroplasts DNA are capable of self-duplication.
[VII] Chloroplast ribosomes :
Lyttleton (1962) isolated chloroplast ribosomes, which are estimated to make up 3 to 7% of the chloroplast dry mass. Chloroplast ribosomes are smaller than cytoplasmic ribosomes. These are 60-66S. Chloroplast ribosomes also dissociate reversibly into 50S and 35S subunits, in a way that is found in E. coli ribosomes (Boardman et al., 1966). Chloroplasts have three types of RNA required for protein synthesis: ribosomal, transfer and messenger. Chloroplast ribosomes associate to form polysomes for synthesis of proteins (Gunning and Steer, 1975).
[VIII] Protein synthesis :
Protein synthesis in mitochondria and chloroplasts is similar to that of prokaryotes. For example, the size of chloroplast ribosomes is the same as ribosomes of blue-green algae, and ribosomes of chloroplasts and mitochondria more closely resemble prokaryotic ribosomes in antibiotic sensitivity than they do eukaryotic ribosomes.
Related Articles:
- Differences between Respiration and Photosynthesis
- Photochemical and Biosynthetic Phase of Photosynthesis
- Photosynthesis
- Anybody can ask a question
- Anybody can answer
- The best answers are voted up and rise to the top
Forum Categories
- Animal Kingdom
- Biodiversity
- Biological Classification
- Biology An Introduction 11
- Biology An Introduction
- Biology in Human Welfare 175
- Biomolecules
- Biotechnology 43
- Body Fluids and Circulation
- Breathing and Exchange of Gases
- Cell- Structure and Function
- Chemical Coordination
- Digestion and Absorption
- Diversity in the Living World 125
- Environmental Issues
- Excretory System
- Flowering Plants
- Food Production
- Genetics and Evolution 110
- Human Health and Diseases
- Human Physiology 242
- Human Reproduction
- Immune System
- Living World
- Locomotion and Movement
- Microbes in Human Welfare
- Mineral Nutrition
- Molecualr Basis of Inheritance
- Neural Coordination
- Organisms and Population
- Plant Growth and Development
- Plant Kingdom
- Plant Physiology 261
- Principles and Processes
- Principles of Inheritance and Variation
- Reproduction 245
- Reproduction in Animals
- Reproduction in Flowering Plants
- Reproduction in Organisms
- Reproductive Health
- Respiration
- Structural Organisation in Animals
- Transport in Plants
- Trending 14
Privacy Overview
Home — Essay Samples — Science — Photosynthesis — Why Photosynthesis Is Essential To Life On Earth

Why Photosynthesis is Essential to Life on Earth
- Categories: Photosynthesis
About this sample

Words: 326 |
Published: Feb 12, 2019
Words: 326 | Page: 1 | 2 min read
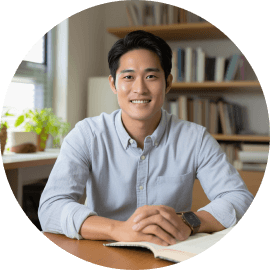
Cite this Essay
Let us write you an essay from scratch
- 450+ experts on 30 subjects ready to help
- Custom essay delivered in as few as 3 hours
Get high-quality help
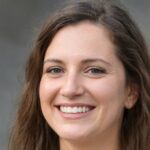
Verified writer
- Expert in: Science
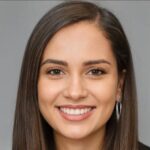
+ 120 experts online
By clicking “Check Writers’ Offers”, you agree to our terms of service and privacy policy . We’ll occasionally send you promo and account related email
No need to pay just yet!
Related Essays
1 pages / 506 words
1 pages / 522 words
3 pages / 1142 words
1 pages / 407 words
Remember! This is just a sample.
You can get your custom paper by one of our expert writers.
121 writers online
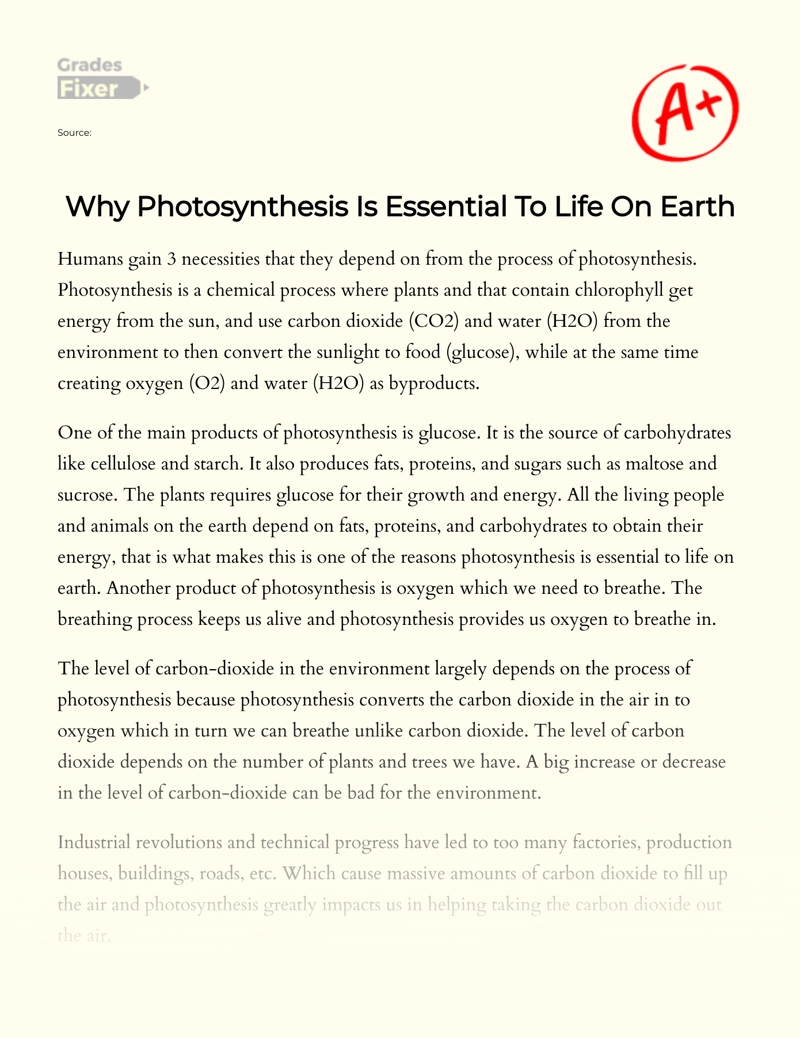
Still can’t find what you need?
Browse our vast selection of original essay samples, each expertly formatted and styled
Related Essays on Photosynthesis
Photosynthesis, the process by which green plants and certain other organisms transform light energy into chemical energy. During photosynthesis in green plants, light energy is captured and used to convert water, carbon [...]
Photosynthesis happens in the light. Cellular respiration happens in the dark. In the process of photosynthesis, the plants need sunlight for energy. Photosynthesis is the process that plants use light energy from the sun to [...]
In this experiment, we were testing the rate of photosynthesis in elodea. For a plant to photosynthesize, it needs carbon dioxide and water and sunlight, a factor of photosynthesis. In order for us to measure the rate of [...]
We as heterotrophs rely on photosynthetic organisms for nearly all the organic plant matter that we consume for energy. Photosynthesis is one of the oldest and one of the most fundamental processes of life. ("BIO 1510 Laboratory [...]
Many illnesses are hereditary; for instance, Huntington's and Cystic Fibrosis. Other illnesses affected by genes, such as diabetes and cancer. Many advancements make it workable for a solution to target such hereditarily [...]
There are many different processes that happen within the carbon cycle. Photosynthesis is one of these processes that happens everywhere on Earth. The reaction occurring during photosynthesis, with the addition of solar energy, [...]
Related Topics
By clicking “Send”, you agree to our Terms of service and Privacy statement . We will occasionally send you account related emails.
Where do you want us to send this sample?
By clicking “Continue”, you agree to our terms of service and privacy policy.
Be careful. This essay is not unique
This essay was donated by a student and is likely to have been used and submitted before
Download this Sample
Free samples may contain mistakes and not unique parts
Sorry, we could not paraphrase this essay. Our professional writers can rewrite it and get you a unique paper.
Please check your inbox.
We can write you a custom essay that will follow your exact instructions and meet the deadlines. Let's fix your grades together!
Get Your Personalized Essay in 3 Hours or Less!
We use cookies to personalyze your web-site experience. By continuing we’ll assume you board with our cookie policy .
- Instructions Followed To The Letter
- Deadlines Met At Every Stage
- Unique And Plagiarism Free
We think you are located in South Africa . Is this correct?
- Yes, I reside in South Africa
- Change country/curriculum
We use this information to present the correct curriculum and to personalise content to better meet the needs of our users.
1.1 Photosynthesis
Photosynthesis and respiration.
Chapter overview
This chapter provides learners with an introduction to, and revision of, the concepts in photosynthesis and respiration in preparation for a study of the ecosystem. Learners have already looked at photosynthesis and respiration in previous grades. They know that respiration is one of the seven life processes of living things. They have also been introduced to photosynthesis in the context of green plants and food chains in Gr. 5 and 6. The emphasis in this chapter is on the use of energy and on how radiant (light) energy is transferred to chemical potential energy and later released during respiration. This concept is developed within the scope of the CAPS prescriptions and will be used as a scaffold to explain the transfer of energy in the ecosystem in the subsequent chapter. As an introduction teachers may want to watch or show the following video to the class:
These tables and how to use them are explained in the Teachers' Guide Overview at the front of the book. We have also explained how to use the bit.ly links to websites and videos in the front of the book.
1.1 Photosynthesis (3.5 hours)
1.2 Respiration (2.5 hours)
- What drives life on Earth and in ecosystems?
- How do green plants photosynthesise when no other organism can make its own food?
- What do plants do with the food that they produce?
- Why do we need to eat food? What does it provide us with?
- We know respiration is one of the seven life processes, but what happens during respiration in organisms?
Energy is needed to sustain life and without it nothing would be able to live on Earth. Our most important source of energy is the Sun. In this chapter we are going to investigate the processes involved in transferring the Sun's energy to our bodies to allow us to read this text! These two important processes are photosynthesis and respiration .
Photosynthesis
- chemical potential energy
- chlorophyll
- chloroplast
- photosynthesis
- radiant energy
- respiration
This website has many interesting articles about science and science related jobs. They have been classified according to topics and also provide tips on how to incorporate the articles into your classroom. If you are interested in incorporating real world science into your classroom, this is one website to start looking at: http://www.sciencenewsforkids.org/news-in-the-classroom/
All the New words listed in the boxes in the margin are defined in the glossary at the end of this strand.
Energy sustains life
All life on Earth depends on energy to sustain the seven life processes.
The seven life processes
INSTRUCTIONS:
- Do you remember what the seven life processes are? Do you remember using the letters from MRS GREN to help you remember these?
- Write down the seven life processes below.
The seven life processes are: movement (moving), reproduction (reproducing), sense the environment (sensing), growth (growing), respiration, excretion (excreting), nutrition (feeding).
The form of energy that the Sun produces is called radiant energy . Although the Sun provides us with both light and warmth, plants only use the light energy from the Sun to photosynthesise.

Most organisms cannot directly use the energy from the sun to perform the seven life processes. For example, a reptile can lie in the Sun to warm up from the heat energy, but this does not provide the necessary energy for that animal to move, reproduce or excrete waste.
Except for a few sea slugs, plants are the only organisms on Earth that can absorb the Sun's radiant energy and convert it into food for themselves and for other living organisms.
Radiant energy to chemical potential energy
The Visit boxes in the margins contain links to interesting websites and videos. Simply type the link exactly as it is into the address bar in your browser.
What is potential energy? Do you remember that we spoke about energy for movement (kinetic energy) and energy that is stored (potential energy) in Energy and Change in Gr. 6 and 7? What are some things that have kinetic energy and some that have potential energy? Remember to take down some notes in the margins of your workbook as you discuss things in class.
Ask your learners this as a revision of what they did in previous grades. Some objects with kinetic energy are a moving car, a bouncing ball, a leaf blowing, a fan blowing, etc. Some objects with potential energy are a book on the table (it has gravitational potential energy as it can fall down to the ground), a bouncing ball when it is at the top of its bounce as it can also fall back down, a batteries, fossil fuels have and food have potential energy.
All living organisms can use energy in the form of chemical potential energy for the life processes. This is the energy that is stored in the food that organisms eat. Plants are able to capture the radiant energy from the Sun and transfer it to chemical potential (stored) energy for other organisms to use. They do this through the process of photosynthesis. All organisms release the stored potential energy from the food that they eat to support their life processes. This process is called respiration .
Find out why plants that photosynthesise are green.
Photosynthesis takes place in small structures called chloroplasts , which are inside the cells of the leaves and stems of green plants. Inside the chloroplasts are green pigments called chlorophyll .This is what gives plants their green colour. Photosynthesis is the process in which chlorophyll molecules absorb the radiant energy from the sun and transfers it into chemical potential energy. The only function of chlorophyll is to trap the sunlight energy; chlorophyll is not produced or used up during photosynthesis.
Chloroplasts are a type of organelle found only in plant cells. A cell is the basic unit of all living things. We will learn more about the structure and functioning of cells next year in Gr. 9.
Chloroplasts are only present in plants. However some sea slugs have learnt to absorb the chloroplasts from the green algae that they eat into their bodies and can actually photosynthesise themselves!

Photosynthesis has other requirements besides light energy from the Sun. What are these? Look at the following diagram which summarises the process of photosynthesis.

Plants use radiant energy from the Sun in a series of chemical reactions to change carbon dioxide from the air and water from the soil into glucose . The process releases oxygen.
Requirements and products of photosynthesis
- Summarise what you have learnt about photosynthesis in the diagram below.
- Fill in the requirements of photosynthesis in the block on the left and fill in what type of energy is needed and the name of the pigment that absorbs the energy.
- Fill in the products of photosynthesis in the block on the right.

The learner's diagram should look as follows:

The process of photosynthesis can be presented in the form of an equation:

What happens to the glucose that plants produce during photosynthesis?
Glucose storage and use
The glucose that a plant produces when it photosynthesises is the food for the plant. The plant can use this glucose directly, and release the energy during its own respiration or it can store the glucose or convert it into other chemical compounds.
Glucose is soluble in water. As we learnt in Matter and Materials in Gr. 6, this means that glucose can dissolve in water. This is useful to the plant as it means it can transport the glucose in water to where it is needed elsewhere in the plant. However, in order to store large amounts of glucose, plants need to convert it into compounds which are insoluble in water. Therefore the plant converts glucose into starch , which is insoluble in water. Why do you think the plant might need to store some glucose?
Discuss this with your learners. The glucose is produced continuously during the day when the Sun is out and is not all used at once by the plant. The plant cannot have large amounts of glucose accumulating as this affects the water potential within the leaves, and so some is converted to starch to be stored until it is needed.
In addition to starch, plants also convert glucose into cellulose. Cellulose is used to support and strengthen plants. Animals do not have cellulose for support. Instead animals have something else to provide support and protect the body. Do you remember what this is?
A skeleton.
Glucose is also converted into other chemical compounds that enable processes in the plant such as reproduction and growth.
Plants use sugars (glucose) as a basic molecule from which to make hundreds of other compounds, such as proteins, oils, vitamins, colourful pigments in flowers, strong tasting chemicals (hot chilli plant), sweet tasting nectar and sweet smelling fragrances.
We have now learnt about how plants produce glucose and store it as starch, but how do we know for sure? As young scientists we also need to question whether this explanation of photosynthesis is accurate. Is there an investigation we can do to test for the presence of these compounds? Let's find out!
We have learnt that plants produce glucose during photosynthesis and store this in the form of starch. Therefore, to see if a plant photosynthesises, we can test to see if the plant produced starch.
Study the following properties of starch and glucose with your class. Think of possible tests that can be done to determine whether a plant has produced either starch or glucose. Record some of your discussion points.
Discuss this as a class in the lead up to the investigation.
- Glucose tastes sweet but starch does not taste sweet at all.
Learners could suggest that they taste the substance to see if it is a starch or glucose. It is important that learners be made aware that we do not taste-test unknown substances due to the potential for poisoning. This specific point was included to allow teachers to reinforce this rule with learners and that we only ever do a taste test if we are sure that a substance is in fact edible.
- Glucose will dissolve in water while starch will not dissolve in water.
Although this can be used as a fairly simple, physical test, it has several problems. For example, the temperature of the water will affect the rate at which it dissolves, as will the quantities used, etc. In discussing these points facilitate the discussion and lead learners to conclude it is not a very accurate test, although a simple physical one that can give a fairly good indication. This is a good opportunity to discuss kinetic energy and temperature of a solution.
- Iodine changes from brownish-orange to dark blue-black when it comes into contact with starch. Have a look at the following photos which illustrate this.
This is included as an introduction to the subsequent investigation. Teachers should let learners explore the possible ways in which to use this to test for starch.

Please emphasise to learners that they should refer to iodine solution , and not just iodine (which is a bluish black solid).
A video on the starch test.
Now that we know that plants produce glucose and change this into starch, we can find out if all leaves produce the same amount of starch through photosynthesis.
Which leaves photosynthesise?
You will need variegated and normal leaves for this investigation. Variegated leaves have white patterns (areas lacking chlorophyll) on them. There are many examples of South African plants that have variegated leaves, such as some geraniums, African violets, ivy, etc. You can also take a walk around your school property and surrounds to see if you can find any variegated leaves. Get learners to look at the leaves and discuss the investigation first. They can also do this in groups. This investigation can be done over 2 lessons. You should place one set of pot plants in the cupboard the day before you want to do part 1 of the investigation. After you have done part 1, you can do part 2 in the following lesson. In part 2, learners will need to write up an experimental report. If you do not have time to do both parts of the investigation, a suggestion is to get your learners to read through Part 1, and then to conduct Part 2 where they have to write up their own report.
There are two parts to this investigation. First, we want to find out which leaves are able to photosynthesise. We will place some pot plants in the light for a day, and some other pot plants in a dark cupboard for a day, and then perform the investigation on the leaves of plants from both groups.
In the second part of the investigation, we will use what we have learnt to investigate which parts of variegated leaves photosynthesise.
Part 1: Leaves in light and dark
What do you wish to establish by conducting this investigation?
Learner-dependent answer
Possible answers include: 'To determine whether leaves photosynthesise in the dark or the light', or 'To investigate whether Light is necessary for Photosynthesis'.
HYPOTHESIS :
What do you think or predict will happen when you conduct this investigation?
The leaves in the light will test positive for starch as they photosynthesised, whereas the leaves in the dark will not photosynthesise and will test negative for starch.
MATERIALS AND APPARATUS :
- a range of pot plants that can be easily moved around
- 100 ml beaker or glass jar in a saucepan with water
- bunsen burner, spirit lamp or a stove
- ethyl alcohol (or methylated spirits)
- glass petri dishes, white saucer or white tile
- stopwatch or timer
- glass pipette or dropper
- iodine solution
Before starting this investigation, all plants must be placed in a dark cupboard for up to 48 hours prior to starting the investigation in order to ensure that the plants do not have starch in the leaves before the process begins.
- Work in groups of three or four.
- Place half of the plants in the dark for at least 24-48 hours and the others in a well-lit area of the class that is exposed to lots of natural sunlight.
- After 24 hours, pour 50 ml of the ethyl alcohol into the beaker and place it in the saucepan with water. Heat the saucepan over the bunsen burner or the stove. The water in the saucepan will distribute the heat evenly to warm the ethyl alcohol evenly.
Instead of a saucepan with water and a beaker with alcohol, teachers may use a beaker with water and a test tube with meths or alcohol. As long as the container holding the alcohol is safely contained within the water to prevent it from being directly heated or coming into contact with the flame. Teachers may also feel safer if they demonstrate this experiment.
- Remove one healthy looking leaf from the pot plants that were in the well-lit area exposed to direct sunlight.
- Using the tweezers, dip a leaf into the boiling water for 1-2 minutes. This helps to remove the waxy cuticle that covers the leaf and breaks down the cell walls.
- After this, place the leaf into the beaker with the ethyl alcohol.
Warning: The alcohol needs to be heated as well but it cannot be heated directly because it is extremely flammable. It therefore needs to be heated in a water bath.
- Leave the leaf in the alcohol until all the chlorophyll has been removed from the leaf and the alcohol turns green.
- Place the leaf into warm water to soften it.
- Remove the leaf from the warm water and place it on a white tile or a petri dish on top of a white surface.
- Use the pipette or dropper to carefully drop 2 or 3 drops of iodine solution on the leaf in the petri dish and record your observations.
The amount of iodine solution required to observe a result depends on the leaf tested. Some leaves may need to be flooded with iodine solution. Place the leave in a petri dish and cover the leaf with iodine.
- Repeat this process for two more leaves that were in the well-lit area.
- Remove the plants that were in the dark for at least 24 hours. Use the test above to test whether there is starch present in the leaves from the plants that were kept in the dark.
- Record your observations.
A really good demonstration of the various experiments in this and subsequent sections is found at . It starts off with carbon dioxide through limewater test and then demonstrates the starch test.
RESULTS AND OBSERVATIONS :
Keep a record of your observations. Draw a table to record and compare your results.
Learners must draw their own tables to record their observations. This being the first investigation that they will perform in high school, this activity will allow teachers to gauge their level of proficiency and abilities in this regard.
The hot water removes the waxy cuticle and the alcohol dissolves the chlorophyll, releasing the green colour of the leaf. After the leaves are taken from the ethyl alcohol they should be white. The chlorophyll needs to be removed from the leaf so it does not mask the colour change we expect with the iodine solution. When iodine is dropped on the leaf it turns blue-black in the presence of starch.This is an indicator to show that the leaf was photosynthesizing and producing glucose that was turned into starch.
CONCLUSION :
What did you learn from doing this investigation?
Plants that were given sunlight were able to photosynthesise and therefore tested positive for starch (iodine solution turned blue-black). Those that did not receive radiant energy did not photosynthesise so they tested negative for starch presence (iodine solution remained yellow-brown). Therefore, light is necessary or required for photosynthesis to occur.
QUESTIONS :
Why were some plants placed in a well-lit area with direct sunlight and others in the dark?
This was done to allow some plants to photosynthesise and the others not. Thus those that were able to photosynthesise could produce starch and those that didn't only had small amounts of starch or no starch.
Explain what the results of the iodine test indicates.
If the iodine changes from brownish-orange to dark blue-black that indicates that the leaf or other part of the plant contains starch and must have photosynthesised. If the iodine solution does not change colour then the leaf or other part of the plant does not contain starch and did not photosynthesise.
Part 2 : Which parts of variegated leaves photosynthesise?
Learners need to apply what they have learnt in Part 1 to plan their own experiment in part 2 and write up an experimental report.
Have a look at the following photos of different plants. What do you notice about the leaves?

Geranium leaves are an excellent option for this investigation. The ivy has a very thick cuticle and the iodine can't penetrate the leaf easily. Variegated mint-leaf is also very good for this investigation, and is a common plant in Kwa-Zulu Natal.
We call these leaves variegated as they have green and white sections. We want to find out which parts of these leaves photosynthesise in this part of the investigation.
- You need to design this investigation yourself.
- First decide what question you are trying to answer and the aim of your investigation.
- Make a hypothesis for your investigation.
- You then need to think back to part 1 and design the method for your investigation.
- After conducting the investigation, you need to write up an experimental report of your findings.
- Materials and apparatus
- In your results section you need to record your observations in a scientific way. You can do this using a table, diagrams or a combination of both. Think carefully about what information you need to record in order to come to conclusions at the end of your experiment.
- In your discussion, you need to explain your results and what they mean. You also need to evaluate your investigation and explain if there were any unusual results and suggest ways that you could have improved your investigation for future researchers who might want to repeat what you have done.
- Present your report on separate paper.
This activity may be done in groups or individually. Up until now learners have been provided with a framework in which to write up their results. For this investigation, they need to compile their own reports. Some examples of what learners might produce are included here:
Aim: To find out which parts of variegated leaves photosynthesise and store starch.
Hypothesis: The green parts of variegated leaves will turn blue-black in iodine solution indicating that they photosynthesise and store starch, whereas the white parts will not turn blue-black (will stay brown).
Materials and apparatus: This should be a similar list to what was used in Part 1. Learners must record the items in a bulleted list and take note of measurements.
Method: This should also be similar to Part 1. The steps in the method must be numbered. They must be written in full sentences. Learners must take note of what measurements they used.
Results: Learners should draw a table to record their results. They should provide headings for the columns and rows and also a heading for the table. Learners must make drawings of their leaves at the start of the experiment, indicating the different coloured regions as boiling in alcohol will remove the colour. They can then indicate the results on the drawings.
Discussion: Assess learners ability to explain their results. They should also make reference to the fact that the white parts of the leaves do not contain chlorophyll and therefore they do not photosynthesise. This also shows that chlorophyll is crucial for photosynthesis. Learners should explain anything that they might have improved on in their results.
Conclusion: This should be a short statement in which they answer their aim or investigative question.
Leaves are not the only parts of plants that store starch. Starch is also stored in the stems, roots and fruit. Have you ever wondered why fruit becomes sweeter as it ripens? Think of an unripe green banana and a ripe yellow banana. Which one is sweeter? Let's find out why.
Why do bananas become sweeter as they ripen?
It is not crucial to do this investigation if you do not have time. This is an optional extension of the starch test.
In this investigation we will taste the bananas to determine if they have more glucose or more starch. We will also conduct a starch test on the ripe and unripe bananas to see which contain more starch.

An example of a possible answer is: To investigate the presence of starch in ripe and unripe bananas; To investigate why bananas becomes sweeter as they ripen; etc.
If the unripe banana does not taste so sweet compared to the very sweet tasting ripe banana then perhaps it contains more starch, and less glucose. The starch test might show that the unripe banana contains more starch.
- ripe and unripe bananas cut into discs
- petri dish or saucer
Work in groups of three or four. Take a piece of the ripe banana and a piece of the unripe banana and compare the tastes and textures of each. Record your observations in a table. Which banana do you think contains the most starch and the least glucose (a sugar) based on the taste test?
Learners should be able to describe the taste and texture of each banana: the ripe one being soft and sweet, and the unripe one being firm and not too sweet. Learners might say that the unripe banana contains more starch and less glucose as it is not as sweet as the ripe banana. But, this is not a very accurate test. The learners might also experience the tastes differently, making the results unreliable.
- Use the iodine starch test identify which banana, the ripe or the unripe one, contains the most starch. Record your observations in the table.
Learners should be able to quite easily see this based on the speed with which the iodine changes colour in the unripe banana.
- Compare this test to the results from your taste and texture test to identify which banana contained the most starch.
OBSERVATIONS:
Draw a table to record your observations from the taste and iodine test for starch.
Allow learners the freedom to tabulate their results in any way they choose as long as it is easy to interpret and understand. Use this as a teaching opportunity to help those who cannot do this and when everyone is done compare the different methods used in the class.
Compare your observations of ripe and unripe bananas with those of the other learners in the class. Did you all make the same observations?
Learner-dependent answer.
You should use this comparison to help learners understand that reliability of an experiment rests on the fact that although different people perform the same test they should all reach very similar results.
What do you conclude from these results? Which method of testing is better to use and why do you say so?
The conclusion is that unripe bananas contain more starch than ripe bananas. This question was specifically included to introduce learners to the concept of validity and teachers are encouraged to allow learners to debate this issue in the class.
Explain what you think happens to the starch as the bananas ripen.
As the bananas ripen, the starch is converted into glucose.
Now that we have looked at how green plants produce their own food, let's find out how all living things release the energy stored in food in order to perform the life processes.
Respiration
The term respiration can refer to two distinct processes. In physiology , respiration refers to the transport of oxygen from the outside air to the cells and the transport of carbon dioxide out of the tissues and into the air. This is often confused with breathing, which is the movement of air in and out of the breathing organs, such as lungs or gills, and does not take place in all organisms, whereas respiration does. At a biochemical level, respiration refers to cellular respiration. This is the metabolic process in all organisms where oxygen is combined with glucose to release water and carbon dioxide and energy in the form of ATP (adenosine triphosphate). Cellular respiration takes place in individual cells within the organism whereas physiological respiration involves the bulk transport of gases and other compounds between the organism and the outside air.
In this section, we will be looking specifically at cellular respiration as we will be looking at the chemical reactions which release the energy in food. However, learners have not yet learnt about cells and so we will just refer to this as respiration. Should you wish to do so, you can make this distinction to your learners and introduce the term cellular respiration, however, it will only be clear once they have done cells in Gr. 9. They will study physiological respiration in Gr. 9 when they do body systems and also cover the circulatory and respiratory systems in detail.
An idea as introduction and/or conclusion to this section on Respiration is to let learners watch the YouTube video of a song about cell respiration: bit.ly/1eupZUz . The initial explanation is simple and appropriate for this grade level but it might show some of the more inquisitive learners what actually happens during the 3 stages of respiration.
We have now seen how plants produce food during photosynthesis. The energy from this food needs to be used by plants and by all the animals who eat those plants. In fact, all organisms need to break down food in order to release its chemical potential energy for life processes. So how does this happen? Let's find out.
Energy from food
Our bodies need energy to move and do work. Where do we get our energy from? The energy is obtained from the food that we and all other organisms eat.
If you think back to the work you did on fuel and energy in previous grades in Energy and Change, you will remember that fuels, such as wood, coal, and oil, contain chemical potential energy . When this fuel is burned in the presence of oxygen, the chemical potential energy is transferred into light and heat energy. In the same way, the glucose from the food that you eat is combined with oxygen in a series of chemical reactions to release the energy. The glucose is broken down and the energy is released. This energy is then used to drive all the other processes in your body. This process is called respiration . We can define respiration in all living organisms as the process by which energy is released from glucose in a series of chemical reactions.
We will learn more about chemical reactions next term in Matter and Materials. You will also learn more about respiration in later grades.
Respiration takes place in all organisms, even plants. However, plants do not need to eat any food as they make their own food during photosynthesis.
Photosynthesis requires sunlight and can only take place during daylight hours. Learners may get confused and think that because photosynthesis occurs during the day, that respiration only occurs during the night. Ensure that learners understand that respiration occurs constantly, during the day and night.
Products of respiration
Do you remember how we represented photosynthesis as an equation to show what goes in and what comes out? We can represent respiration as an equation in the same way as we did for photosynthesis.
We know what is required for respiration to take place in all organisms. List the two ingredients for respiration.
They are glucose (food) and oxygen. Next term in Matter and Materials, we will look at chemical reactions and define the 'ingredients' as reactants. You can also link back to this example of a chemical reaction (actually a series of reactions), when introducing the topic next term.
We also know that respiration releases energy. This energy is contained in the chemical bonds of ATP molecules. ATP is not energy itself, but instead it stores energy. The ATP molecules have chemical potential energy in their bonds. When the ATP molecules are broken down they release the energy in order for other processes to take place.
However, respiration does not only produce energy. It also produces water and carbon dioxide as by-products. We can write an equation for respiration as follows:
glucose + oxygen → carbon dioxide + water + energy
Unlike photosynthesis, where we place sunlight energy above the arrow and not as one of the reactants in the equation, in respiration, we place energy here as a product. This is because the energy is actually contained within the molecules produced (ATP). However, at this level learners do not need to know this term, and we will only use the word energy in the equation. However, since this has the potential to cause misconceptions later, a note was included about the energy being contained within ATP molecules.
During photosynthesis in plants, oxygen is produced as a by-product. We call it a by-product as it is not the main product that is wanted from the process. In photosynthesis, the main product that is required from the process is glucose. What are the by-products in respiration?
Ask your learners this question to see if they understand the concept. The by-products are carbon dioxide and water.
A by-product is also sometimes referred to as a waste product.
The carbon dioxide that is produced in the body of an organism during respiration needs to be removed. In humans, we do this by breathing out carbon dioxide-rich air. We will learn more about the whole respiratory system next year in Gr. 9, and how breathing, our blood circulation system and respiration all work together as one system within our bodies.
Do not confuse breathing with respiration! Breathing is the act of inhaling and exhaling air into and out of the lungs. Respiration is the metabolic process that uses oxygen to release energy and releases carbon dioxide as a by-product.
We can test for the products of respiration using our own breath. So how do we test that our breath contains carbon dioxide? It is a colourless gas, so we cannot see it directly.
There is a very well known test for detecting carbon dioxide using clear limewater. To test if a gas contains carbon dioxide, simply bubble the gas through limewater . If the clear limewater turns milky, then the gas contains carbon dioxide. Next term in Matter and Materials, we will look at this again and find out about the chemical reaction taking place in the test. For now, let's use this test to show that our breath contains carbon dioxide.
Does our breath contain carbon dioxide?
Watch a video of this activity.
You will need to prepare limewater prior to this activity. Here are instructions on how to do this:
- Place a few tablespoons of calcium hydroxide, Ca(OH) 2 , in a clear 500 ml reagent bottle and fill with water. Shake or stir to make a cloudy suspension.
- Leave the suspension to settle for a few days. The clear liquid above the solid Ca(OH) 2 is a saturated solution of Ca(OH) 2 , also known as clear limewater.
- Carefully decant as much of this as you need, without stirring up the solid Ca(OH) 2 sludge at the bottom.
- To make more, simply add more water, shake it up and let it settle again. When the sludge dissolves completely, add more solid Ca(OH) 2 .
- small beakers (or test tubes)
- rubber tubes or drinking straws
For health reasons, there should be one straw or rubber tube per learner.
- 20 ml syringe (or larger if available)
Pharmacies are generally quite helpful and will assist schools in purchasing low cost syringes. Technology teachers at your school might also have syringes for their syringe mechanics lessons. An alternative to a large syringe is to use a bicycle pump or balloon blower. Safety warning: remove the needles from the syringes, if there are any, before you hand out the syringes in class.
INSTRUCTIONS
- Work in groups of three.
- Mark one beaker AIR and the other BREATH.
- Pour clear limewater into each beaker until they are half full.
- Blow bubbles through the rubber tube into the beaker marked BREATH, as shown in the diagram. Do this for at least 1 minute. Notice what happens to the clear limewater.

- Attach a rubber tube to the front of a syringe. Draw air into the syringe from the atmosphere.
- Place this rubber tube into the beaker marked AIR and push out the air inside the syringe slowly and carefully into the limewater as shown in the diagram. Notice what happens to the clear limewater.

Describe what you observed when you blew air from your lungs through the limewater. What does this mean?
The clear limewater turned cloudy white. This means our breath contains carbon dioxide from respiration.
Describe what you observed when you used the syringe to bubble air from the atmosphere through clear limewater.
The clear limewater did not change and remained clear. Some might notice a very slight change, which indicates that there is a small amount of carbon dioxide present in atmospheric air.
A very small percentage of atmospheric air is carbon dioxide gas (0.03). Why do you think you observed the result you did when you pushed air from the atmosphere through the limewater?
As air contains such a small percentage of carbon dioxide, there is not enough to cause a noticeable difference in the limewater. Discuss with your learners why they think that the limewater turned cloudy with your breath but not with the air, even though air does contain carbon dioxide. Point out that air from your lungs contains a much higher percentage of carbon dioxide from respiration and so this is able to turn the limewater cloudy in a shorter time than atmospheric air will.
Think about respiration.
- What are the requirements for respiration?
- What are the products of respiration?
- Glucose and oxygen.
- Energy, carbon dioxide and water.
Requirements and products of respiration
- Summarise what you have learnt about respiration in the summary diagram below.
- Fill in the requirements of respiration in the block on the right.
- Fill in the products of respiration in the block on the left.

This is what learners' diagrams should look like:

Have you noticed the VISIT boxes in the margins which contain links? You simply need to type this whole link into the address bar in your internet browser, either on your PC, tablet or mobile phone, and press enter, like this:

It will direct you to our website where you can watch the video or visit the webpage online. Be curious and discover more online at our website!
- The need for energy drives the interactions and interdependence in an ecosystem.
- The Sun provides energy to the Earth in the form of radiant (light) energy and heat energy.
- Photosynthesis is the process whereby green plants use carbon dioxide from the air, water from the soil and radiant energy from the Sun in a series of chemical reactions to produce glucose (food) and oxygen.
- Plants are able to photosynthesise because they contain chlorophyll, a green pigment that can capture light energy from the Sun.
- Plants change the glucose that they produce into starch that can be stored more easily.
- Plants also produce cellulose fibres that give plants strength and support and are important to our digestive systems as roughage.
- The food that a plant produces is used by animals when they eat the plant and by other animals that eat them.
- This food contains chemical potential energy that needs to be released from the food.
- Respiration is the process in all living organisms by which energy is released from glucose in a series of chemical reactions.
- Respiration uses oxygen while carbon dioxide and water are given off as by-products.
Concept map
Throughout this year, we are going to develop the skill of designing and making concept maps in Natural Sciences. The "Key concepts" listed above is a summary written out in full sentences. A concept map provides another way of representing information (ideas and concepts) in a more visual way. The benefits of a concept map are that it allows one to show the links between different concepts. Often a concept map has a "focus question" from which the other concepts radiate. In these books, the focus question will be the main topic for the chapter. The relationships between different concepts are shown using arrows with linking phrases, such as "results in", "includes", "can be", "used to", "depends on", etc.
As this year progresses, learners will have to start filling in more parts of the concept maps themselves, and then hopefully draw their own ones by the end of the year. This teacher's guide contains the full version of each concept map. Encourage your learners to study the concept maps and make sense of them at the end of each chapter before doing the revision questions. Help your learners to understand and "read" the concept maps by constructing sentences from them. For example in this case you could read: "Respiration takes place in all organisms, releases energy from food".
Learners need to learn how to learn! This is one skill which might help them later in their school career where they have a lot more information to ingest and learn and make sense of. Concept mapping is one tool for summarising information and understanding how different concepts link together. Real understanding and knowledge comes from grappling with the subject matter, and not just memorizing facts.
"Knowledge is real knowledge only when it is acquired by the efforts of your intellect, not by memory." - Henry David Thoreau
This year in Natural Sciences, we are going to learn more about how to make our own concept maps.
In the summary, we first have the "Key concepts" for this chapter. This is a written summary where the information from this chapter is summarised using words. We can also create a concept map of this chapter. This is a map of how all the concepts (ideas and topics) in this chapter fit together and are linked to each other. A concept map gives us a more visual way of summarizing information.
Different people like to learn and study in different ways; some people like to make written summaries, whilst others like to draw their own concept maps when studying and learning. Others like to make things even more visual, using pictures and diagrams to form their summaries. Figuring out the study method that works best for you, and developing these skills is very useful, especially for later in high school and after school!
Have a look at the concept map below for 'Photosynthesis and Respiration'. Do you see that there are some empty spaces? You need to complete the concept map by filling these in. To do this you need read the map from top to bottom and have a look at the concepts which come before. For example, read the concept map as follows, "Respiration takes place in all organisms. All organisms release energy from food, called …........." What type of energy does food contain? Remember, food is the fuel for our bodies. You also need to fill in the three things that plants use to photosynthesise. You need to look at what concepts link from these in order to know where to put each one. Finally, what does photosynthesis release as a by-product? You also need to fill this in.

Teacher's version.
Below is the complete concept map with the answers filled in. Make sure your learners understand what a concept map is and that they have filled in the correct concepts into the empty spaces. Learners might battle to do this, especially to fill in "Potential energy" as the type of energy in food. Help them by reminding them of the two types of energy, namely kinetic energy and potential energy. You can ask them questions such as, "What do plants get from the Sun which is used for photosynthesis?" (Solar energy), "What do plants get from the soil that they use for photosynthesis?" (water), etc.

Revision questions
A Gr. 4 learner wanted to grow some beans and carefully planted them in a yoghurt tub and watered them. He was scared that his little brother would knock his tub over, so he hid the tub in his cupboard.
- Explain what he would have noticed a few days after planting the beans. [2 marks]
- Predict what would have happened after another few days with the beans hidden in his cupboard. [2 marks]
- Explain why you predicted this outcome for his beans. [2 marks]
- What should he have done to make his bean plants grow tall and strong? [2 marks]
- Depending on what he planted the beans in, he might have noticed a root and first leaves forming.
- The young bean plants would have formed small leaves but the plants would slowly start to die.
- The bean plants would die as the leaves would not have enough radiant energy to allow them to photosynthesise and produce glucose for the plants to use to grow and develop.
- He should have put the plants in a place where they would get enough radiant energy (sunlight) to allow them to photosynthesise.
What are the requirements for photosynthesis to occur? [3 marks]
Carbon dioxide, sunlight and water.
A farmer is growing some tomatoes. He heard from his daughter that plants produce glucose during photosynthesis, so he decided to see for himself. However, when he tested the leaves, he did not find much glucose, but he did find a lot of starch present.
- Why did the farmer see this result? [2 marks]
- Describe the test that the farmer conducted to show that the leaf contained starch. [5 marks]
- The plant converts the glucose to starch as it is easier to store. Therefore, the leaves will indicate a high starch content.
- The chlorophyll must be removed by first dipping the leaf into boiling water for 1-2 minutes to remove the waxy top layer. Then the leaf must be placed in alcohol and this is heated over water to remove the chlorophyll. The leaf is then removed and dipped into hot water to soften it as the ethanol will make the leaf brittle. Iodine solution is dripped on it. Iodine changes from orange-brown to dark blue-black in the presence of starch and when it is dripped onto the leaf it changes to dark blue/black indicating that starch is present.
Do plants undergo photosynthesis and respiration all day and all night? Give reasons for your answer. [4 marks]
Plants only photosynthesise during the day, and not at night. This is because they need sunlight energy to photosynthesise. Plants respire all day and all night. All living organisms need to undergo respiration to release energy from chemical potential energy and perform the seven life processes.
A group of Gr. 7 learners wanted to show that carbon dioxide is used to make bread rise because the yeast and sugar that is added to the bread mix produces the carbon dioxide. They set up the following two experiments. The gas that they collected from each test tube was run through limewater.

- Why did they run the rubber tube from Test Tube A to Test Tube B? [3 marks]
- Explain why they added a stopper into the top of Test Tube A. [1 mark]
The following photo shows one of the test tubes after the experiment. Which test tube do you think it is and from which set-up. Give reasons for your answer. [2 marks]

- Why do you think the yeast solution in Set-up 1 did not produce carbon dioxide. [2 marks]
- The learners wanted to collect any gas that formed from the solution in Test Tube A and bubble it through the limewater. If the limewater changed from clear to cloudy white then this would indicate the presence of carbon dioxide. (This question test the learner's ability to follow the logic of the experiment and to link the theory learnt and the practical application of it.)
- The learners did not want to lose any of the gas that might potentially form before it had time to bubble through the limewater. It is also important to make sure that the only thing affecting the limewater was the gas released in the experiment, and not carbon dioxide from the atmosphere. (This question tests the practical understanding of the process that was undertaken.)
- It is test tube B as it contained clear limewater to start which has gone milky. It is from Setup 2 as there is yeast and sugar in test tube A in set-up 2 so the yeast ferments the sugar and releases carbon dioxide which turns the limewater milky.
- The yeast needs to act on the sugar to form the carbon dioxide. When the sugar was not available it could not react and could not produce carbon dioxide.
Study the following diagram and fill in the missing information. [6 marks]

Draw a table in the following space to show the differences between the two processes, photosynthesis and respiration. You table should highlight the differences in requirements, the differences in the products, which organisms the processes takes place in, and when. [8 marks]
An example of a table is given here. (Take not that learners have not yet started to call the starting ingredients reactants)
Table of differences between photosynthesis and respiration
Assessment Rubric 4 can be used to mark this table if you would like a more in depth assessment.
Total [44 marks]
Photosynthesis and the environment
- Published: 14 December 2013
- Volume 119 , pages 1–2, ( 2014 )
Cite this article
- Asaph B. Cousins 1 ,
- Matt Johnson 2 &
- Andrew D. B. Leakey 3 , 4
3218 Accesses
4 Citations
Explore all metrics
Avoid common mistakes on your manuscript.
Nearly 240 years after Joseph Priestley’s influential experiments involving a mouse, a plant and a bell jar the need and desire to study photosynthesis and the environment has not diminished. In fact, it is well recognized that the relationship between photosynthesis and the environment is key to understanding the health of our planet, in addition to providing clean air, water and food security across the globe. Although there is a wealth of information, scaling across time (femtosecond to gigayear) and space (angstrom to globe), on the response of photosynthesis to changing environmental conditions there is still much to be learned about the interaction between photosynthetic processes and the environment in which it happens. In fact this has never been truer as our planet’s climate changes at unprecedented rates and the population of humankind continues to grow (both in number and girth).
With this in mind we present a special issue of Photosynthesis Research entitled, “Photosynthesis and the Environment”, which is a compilation of exciting new work on photosynthesis and the environment at a range of scales from the biophysical and molecular to the physiological and biogeochemical, including evolutionary and ecological perspectives. Integration across these scales and the merging of traditionally distinct approaches are key features of the work. The research ideas presented here come from some of the best early career researchers in photosynthesis whom have received their Ph.D. within 15 years of 2013. We solicited early career scientist for this review issue as they can potentially provide a unique perspective on the future of photosynthesis research. Additionally, these scientists are in the trenches of training the next generation(s) of interdisciplinary scientists as well as engaging the non-scientific community about the importance of both fundamental and applied photosynthetic research.
We’ve organized the structure of this special issue scaling from large to small. The first publications address issues relating to global modeling of photosynthesis (Dietze 2013 ), the use of biochemical parameters to constrain these models (Rogers 2013 ), and the influence of climate (Desai 2013 ) and seasonal changes (Stoy et al. 2013 ) to canopy level photosynthesis. At the physiological level, manuscripts discuss the use of leaf optical measurements (Ainsworth et al. 2013 ), the role of internal CO 2 diffusion (Buckley and Warren 2013 ), the thermal acclimation of photosynthesis (Way and Yamori 2013 ), and the thermal response of different photosynthetic functional types (Yamori et al. 2013 ). Following this, a set of manuscripts addresses integration of photosynthesis with other key processes including water use and respiration; specifically discussing genetic variation in water use efficiency (Easlon et al. 2013 ), the role of redox state on stomatal regulation (Busch 2013 ), and the interaction of mitochondrial metabolism and photosynthesis (Araújo et al. 2013 ). The special features of C 4 photosynthesis are then discussed both in terms of natural variation in C 4 Kranz (Covshoff et al. 2013 ), and single-cell C 4 photosynthesis (Sharpe and Offermann 2013 ). Ultimately, at the molecular and biochemical level, manuscripts address circadian regulation of photosynthesis (Dodd et al. 2013 ), Rubisco (Cavanagh and Kubien 2013 ), Rubisco activase (Mueller-Cajar et al. 2013 ), pigment regulation of light harvesting (Holleboom and Walla 2013 ), pigment biosynthesis (Sobotka 2013 ), thylakoid reactions (Johnson and Ruban 2013 ) and thylakoid organization (Sznee et al. 2013 ).
We are excited about the findings and opinions presented here and the discussion of future research directions collected in these manuscripts. As for many centuries, this is an exciting time to study photosynthesis, and it is clear that this area of research has a bright future that will assimilate much more valuable knowledge as this multidisciplinary field continues to move forward.
Ainsworth EA, Serbin SP, Skoneczka JA, Townsend PA (2013) Using leaf optical properties to detect ozone effects on foliar biochemistry. Photosynth Res. doi: 10.1007/s11120-013-9837-y
PubMed Google Scholar
Araújo WL, Nunes-Nesi A, Fernie AR (2013) On the role of plant mitochondrial metabolism and its impact on photosynthesis in both optimal and sub-optimal growth conditions. Photosynth Res. doi: 10.1007/s11120-013-9807-4
Buckley TN, Warren CR (2013) The role of mesophyll conductance in the economics of nitrogen and water use in photosynthesis. Photosynth Res. doi: 10.1007/s11120-013-9825-2
Busch FA (2013) Opinion: the red-light response of stomatal movement is sensed by the redox state of the photosynthetic electron transport chain. Photosynth Res. doi: 10.1007/s11120-013-9805-6
Cavanagh AP, Kubien DS (2013) Can phenotypic plasticity in Rubisco performance contribute to photosynthetic acclimation? Photosynth Res. doi: 10.1007/s11120-013-9816-3
Covshoff S, Burgess SJ, Kneřová J, Kümpers BMC (2013) Getting the most out of natural variation in C 4 photosynthesis. Photosynth Res. doi: 10.1007/s11120-013-9872-8
Desai AR (2013) Influence and predictive capacity of climate anomalies on daily to decadal extremes in canopy photosynthesis. Photosynth Res. doi: 10.1007/s11120-013-9925-z
Dietze MC (2013) Gaps in knowledge and data driving uncertainty in models of photosynthesis. Photosynth Res. doi: 10.1007/s11120-013-9836-z
Dodd AN, Kusakina J, Hall A, Gould PD, Hanaoka M (2013) The circadian regulation of photosynthesis. Photosynth Res. doi: 10.1007/s11120-013-9811-8
Easlon HM, Nemali KS, Richards JH, Hanson DT, Juenger TE, McKay JK (2013) The physiological basis for genetic variation in water use efficiency and carbon isotope composition in Arabidopsis thaliana . Photosynth Res. doi: 10.1007/s11120-013-9891-5
Holleboom C-P, Walla PJ (2013) The back and forth of energy transfer between carotenoids and chlorophylls and its role in the regulation of light harvesting. Photosynth Res. doi: 10.1007/s11120-013-9815-4
Johnson MP, Ruban AV (2013) Rethinking the existence of a steady-state Δ ψ component of the proton motive force across plant thylakoid membranes. Photosynth Res. doi: 10.1007/s11120-013-9817-2
Google Scholar
Mueller-Cajar O, Stotz M, Bracher M (2013) Maintaining photosynthetic CO 2 fixation via protein remodelling: the Rubisco activases. Photosynth Res. doi: 10.1007/s11120-013-9819-0
Rogers A (2013) The use and misuse of Vc, max in earth system models. Photosynth Res. doi: 10.1007/s11120-013-9818-1
Sharpe RM, Offermann S (2013) One decade after the discovery of single-cell C 4 species in terrestrial plants: what did we learn about the minimal requirements of C 4 photosynthesis? Photosynth Res. doi: 10.1007/s11120-013-9810-9
Sobotka R (2013) Making proteins green; biosynthesis of chlorophyll-binding proteins in cyanobacteria. Photosynth Res. doi: 10.1007/s11120-013-9797-2
Stoy PC, Trowbridge AM, Bauerle WL (2013) Controls on seasonal patterns of maximum ecosystem carbon uptake and canopy-scale photosynthetic light response: contributions from both temperature and photoperiod. Photosynth Res. doi: 10.1007/s11120-013-9799-0
Sznee K, Crouch LI, Jones MR, Dekker JP, Frese RN (2013) Variation in supramolecular organisation of the photosynthetic membrane of Rhodobacter sphaeroides induced by alteration of PufX. Photosynth Res. doi: 10.1007/s11120-013-9949-4
Way DA, Yamori W (2013) Thermal acclimation of photosynthesis: on the importance of adjusting our definitions and accounting for thermal acclimation of respiration. Photosynth Res. doi: 10.1007/s11120-013-9873-7
Yamori W, Hikosaka K, Way DA (2013) Temperature response of photosynthesis in C 3 , C 4 , and CAM plants: temperature acclimation and temperature adaptation. Photosynth Res. doi: 10.1007/s11120-013-9874-6
Download references
Author information
Authors and affiliations.
School of Biological Sciences, Washington State University, Pullman, WA, 99164-4236, USA
Asaph B. Cousins
Department of Molecular Biology & Biotechnology, University of Sheffield, Firth Court, Western Bank, Sheffield, S10 2TN, UK
Matt Johnson
Department of Plant Biology, Institute for Genomic Biology, University of Illinois at Urbana-Champaign, 1402 Institute for Genomic Biology, 1206 W Gregory Dr, Urbana, IL, 61801, USA
Andrew D. B. Leakey
Department of Crop Sciences, University of Illinois at Urbana-Champaign, 1402 Institute for Genomic Biology, 1206 W Gregory Dr, Urbana, IL, 61801, USA
You can also search for this author in PubMed Google Scholar
Corresponding author
Correspondence to Asaph B. Cousins .
Rights and permissions
Reprints and permissions
About this article
Cousins, A.B., Johnson, M. & Leakey, A.D.B. Photosynthesis and the environment. Photosynth Res 119 , 1–2 (2014). https://doi.org/10.1007/s11120-013-9958-3
Download citation
Published : 14 December 2013
Issue Date : February 2014
DOI : https://doi.org/10.1007/s11120-013-9958-3
Share this article
Anyone you share the following link with will be able to read this content:
Sorry, a shareable link is not currently available for this article.
Provided by the Springer Nature SharedIt content-sharing initiative
- Find a journal
- Publish with us
- Track your research

An official website of the United States government
The .gov means it’s official. Federal government websites often end in .gov or .mil. Before sharing sensitive information, make sure you’re on a federal government site.
The site is secure. The https:// ensures that you are connecting to the official website and that any information you provide is encrypted and transmitted securely.
- Publications
- Account settings
Preview improvements coming to the PMC website in October 2024. Learn More or Try it out now .
- Advanced Search
- Journal List
- Portland Press Opt2Pay

Photosynthesis
Matthew p. johnson.
Department of Molecular Biology and Biotechnology, University of Sheffield, Firth Court, Western Bank, Sheffield S10 2TN, U.K.
Photosynthesis sustains virtually all life on planet Earth providing the oxygen we breathe and the food we eat; it forms the basis of global food chains and meets the majority of humankind's current energy needs through fossilized photosynthetic fuels. The process of photosynthesis in plants is based on two reactions that are carried out by separate parts of the chloroplast. The light reactions occur in the chloroplast thylakoid membrane and involve the splitting of water into oxygen, protons and electrons. The protons and electrons are then transferred through the thylakoid membrane to create the energy storage molecules adenosine triphosphate (ATP) and nicotinomide–adenine dinucleotide phosphate (NADPH). The ATP and NADPH are then utilized by the enzymes of the Calvin–Benson cycle (the dark reactions), which converts CO 2 into carbohydrate in the chloroplast stroma. The basic principles of solar energy capture, energy, electron and proton transfer and the biochemical basis of carbon fixation are explained and their significance is discussed.
An overview of photosynthesis
Introduction.
Photosynthesis is the ultimate source of all of humankind's food and oxygen, whereas fossilized photosynthetic fuels provide ∼87% of the world's energy. It is the biochemical process that sustains the biosphere as the basis for the food chain. The oxygen produced as a by-product of photosynthesis allowed the formation of the ozone layer, the evolution of aerobic respiration and thus complex multicellular life.
Oxygenic photosynthesis involves the conversion of water and CO 2 into complex organic molecules such as carbohydrates and oxygen. Photosynthesis may be split into the ‘light’ and ‘dark’ reactions. In the light reactions, water is split using light into oxygen, protons and electrons, and in the dark reactions, the protons and electrons are used to reduce CO 2 to carbohydrate (given here by the general formula CH 2 O). The two processes can be summarized thus:
Light reactions:
Dark reactions:
The positive sign of the standard free energy change of the reaction (Δ G °) given above means that the reaction requires energy ( an endergonic reaction ). The energy required is provided by absorbed solar energy, which is converted into the chemical bond energy of the products ( Box 1 ).
Standard free energy change

Photosynthesis converts ∼200 billion tonnes of CO 2 into complex organic compounds annually and produces ∼140 billion tonnes of oxygen into the atmosphere. By facilitating conversion of solar energy into chemical energy, photosynthesis acts as the primary energy input into the global food chain. Nearly all living organisms use the complex organic compounds derived from photosynthesis as a source of energy. The breakdown of these organic compounds occurs via the process of aerobic respiration, which of course also requires the oxygen produced by photosynthesis.
Unlike photosynthesis, aerobic respiration is an exergonic process (negative Δ G °) with the energy released being used by the organism to power biosynthetic processes that allow growth and renewal, mechanical work (such as muscle contraction or flagella rotation) and facilitating changes in chemical concentrations within the cell (e.g. accumulation of nutrients and expulsion of waste). The use of exergonic reactions to power endergonic ones associated with biosynthesis and housekeeping in biological organisms such that the overall free energy change is negative is known as ‘ coupling’.
Photosynthesis and respiration are thus seemingly the reverse of one another, with the important caveat that both oxygen formation during photosynthesis and its utilization during respiration result in its liberation or incorporation respectively into water rather than CO 2 . In addition, glucose is one of several possible products of photosynthesis with amino acids and lipids also being synthesized rapidly from the primary photosynthetic products.
The consideration of photosynthesis and respiration as opposing processes helps us to appreciate their role in shaping our environment. The fixation of CO 2 by photosynthesis and its release during breakdown of organic molecules during respiration, decay and combustion of organic matter and fossil fuels can be visualized as the global carbon cycle ( Figure 1 ).

The relationship between respiration, photosynthesis and global CO 2 and O 2 levels.
At present, this cycle may be considered to be in a state of imbalance due to the burning of fossil fuels (fossilized photosynthesis), which is increasing the proportion of CO 2 entering the Earth's atmosphere, leading to the so-called ‘greenhouse effect’ and human-made climate change.
Oxygenic photosynthesis is thought to have evolved only once during Earth's history in the cyanobacteria. All other organisms, such as plants, algae and diatoms, which perform oxygenic photosynthesis actually do so via cyanobacterial endosymbionts or ‘chloroplasts’. An endosymbiotoic event between an ancestral eukaryotic cell and a cyanobacterium that gave rise to plants is estimated to have occurred ∼1.5 billion years ago. Free-living cyanobacteria still exist today and are responsible for ∼50% of the world's photosynthesis. Cyanobacteria themselves are thought to have evolved from simpler photosynthetic bacteria that use either organic or inorganic compounds such a hydrogen sulfide as a source of electrons rather than water and thus do not produce oxygen.
The site of photosynthesis in plants
In land plants, the principal organs of photosynthesis are the leaves ( Figure 2 A). Leaves have evolved to expose the largest possible area of green tissue to light and entry of CO 2 to the leaf is controlled by small holes in the lower epidermis called stomata ( Figure 2 B). The size of the stomatal openings is variable and regulated by a pair of guard cells, which respond to the turgor pressure (water content) of the leaf, thus when the leaf is hydrated, the stomata can open to allow CO 2 in. In contrast, when water is scarce, the guard cells lose turgor pressure and close, preventing the escape of water from the leaf via transpiration.

( A ) The model plant Arabidopsis thaliana . ( B ) Basic structure of a leaf shown in cross-section. Chloroplasts are shown as green dots within the cells. ( C ) An electron micrograph of an Arabidopsis chloroplast within the leaf. ( D ) Close-up region of the chloroplast showing the stacked structure of the thylakoid membrane.
Within the green tissue of the leaf (mainly the mesophyll) each cell (∼100 μm in length) contains ∼100 chloroplasts (2–3 μm in length), the tiny organelles where photosynthesis takes place. The chloroplast has a complex structure ( Figure 2 C, D) with two outer membranes (the envelope), which are colourless and do not participate in photosynthesis, enclosing an aqueous space (the stroma) wherein sits a third membrane known as the thylakoid, which in turn encloses a single continuous aqueous space called the lumen.
The light reactions of photosynthesis involve light-driven electron and proton transfers, which occur in the thylakoid membrane, whereas the dark reactions involve the fixation of CO 2 into carbohydrate, via the Calvin–Benson cycle, which occurs in the stroma ( Figure 3 ). The light reactions involve electron transfer from water to NADP + to form NADPH and these reactions are coupled to proton transfers that lead to the phosphorylation of adenosine diphosphate (ADP) into ATP. The Calvin–Benson cycle uses ATP and NADPH to convert CO 2 into carbohydrates ( Figure 3 ), regenerating ADP and NADP + . The light and dark reactions are therefore mutually dependent on one another.

The light reactions of photosynthesis take place in the thylakoid membrane, whereas the dark reactions are located in the chloroplast stroma.
Photosynthetic electron and proton transfer chain
The light-driven electron transfer reactions of photosynthesis begin with the splitting of water by Photosystem II (PSII). PSII is a chlorophyll–protein complex embedded in the thylakoid membrane that uses light to oxidize water to oxygen and reduce the electron acceptor plastoquinone to plastoquinol. Plastoquinol in turn carries the electrons derived from water to another thylakoid-embedded protein complex called cytochrome b 6 f (cyt b 6 f ). cyt b 6 f oxidizes plastoquinol to plastoquinone and reduces a small water-soluble electron carrier protein plastocyanin, which resides in the lumen. A second light-driven reaction is then carried out by another chlorophyll protein complex called Photosystem I (PSI). PSI oxidizes plastocyanin and reduces another soluble electron carrier protein ferredoxin that resides in the stroma. Ferredoxin can then be used by the ferredoxin–NADP + reductase (FNR) enzyme to reduce NADP + to NADPH. This scheme is known as the linear electron transfer pathway or Z-scheme ( Figure 4 ).

The linear electron transfer pathway from water to NADP + to form NADPH results in the formation of a proton gradient across the thylakoid membrane that is used by the ATP synthase enzyme to make ATP.
The Z-scheme, so-called since it resembles the letter ‘Z’ when turned on its side ( Figure 5 ), thus shows how the electrons move from the water–oxygen couple (+820 mV) via a chain of redox carriers to NADP + /NADPH (−320 mV) during photosynthetic electron transfer. Generally, electrons are transferred from redox couples with low potentials (good reductants) to those with higher potentials (good oxidants) (e.g. during respiratory electron transfer in mitochondria) since this process is exergonic (see Box 2 ). However, photosynthetic electron transfer also involves two endergonic steps, which occur at PSII and at PSI and require an energy input in the form of light. The light energy is used to excite an electron within a chlorophyll molecule residing in PSII or PSI to a higher energy level; this excited chlorophyll is then able to reduce the subsequent acceptors in the chain. The oxidized chlorophyll is then reduced by water in the case of PSII and plastocyanin in the case of PSI.

The main components of the linear electron transfer pathway are shown on a scale of redox potential to illustrate how two separate inputs of light energy at PSI and PSII result in the endergonic transfer of electrons from water to NADP + .
Relationship between redox potentials and standard free energy changes

The water-splitting reaction at PSII and plastoquinol oxidation at cyt b 6 f result in the release of protons into the lumen, resulting in a build-up of protons in this compartment relative to the stroma. The difference in the proton concentration between the two sides of the membrane is called a proton gradient. The proton gradient is a store of free energy (similar to a gradient of ions in a battery) that is utilized by a molecular mechanical motor ATP synthase, which resides in the thylakoid membrane ( Figure 4 ). The ATP synthase allows the protons to move down their concentration gradient from the lumen (high H + concentration) to the stroma (low H + concentration). This exergonic reaction is used to power the endergonic synthesis of ATP from ADP and inorganic phosphate (P i ). This process of photophosphorylation is thus essentially similar to oxidative phosphorylation, which occurs in the inner mitochondrial membrane during respiration.
An alternative electron transfer pathway exists in plants and algae, known as cyclic electron flow. Cyclic electron flow involves the recycling of electrons from ferredoxin to plastoquinone, with the result that there is no net production of NADPH; however, since protons are still transferred into the lumen by oxidation of plastoquinol by cyt b 6 f , ATP can still be formed. Thus photosynthetic organisms can control the ratio of NADPH/ATP to meet metabolic need by controlling the relative amounts of cyclic and linear electron transfer.
How the photosystems work
Light absorption by pigments.
Photosynthesis begins with the absorption of light by pigments molecules located in the thylakoid membrane. The most well-known of these is chlorophyll, but there are also carotenoids and, in cyanobacteria and some algae, bilins. These pigments all have in common within their chemical structures an alternating series of carbon single and double bonds, which form a conjugated system π–electron system ( Figure 6 ).

The chemical structures of the chlorophyll and carotenoid pigments present in the thylakoid membrane. Note the presence in each of a conjugated system of carbon–carbon double bonds that is responsible for light absorption.
The variety of pigments present within each type of photosynthetic organism reflects the light environment in which it lives; plants on land contain chlorophylls a and b and carotenoids such as β-carotene, lutein, zeaxanthin, violaxanthin, antheraxanthin and neoxanthin ( Figure 6 ). The chlorophylls absorb blue and red light and so appear green in colour, whereas carotenoids absorb light only in the blue and so appear yellow/red ( Figure 7 ), colours more obvious in the autumn as chlorophyll is the first pigment to be broken down in decaying leaves.

Chlorophylls absorb light energy in the red and blue part of the visible spectrum, whereas carotenoids only absorb light in the blue/green.
Light, or electromagnetic radiation, has the properties of both a wave and a stream of particles (light quanta). Each quantum of light contains a discrete amount of energy that can be calculated by multiplying Planck's constant, h (6.626×10 −34 J·s) by ν, the frequency of the radiation in cycles per second (s −1 ):
The frequency (ν) of the light and so its energy varies with its colour, thus blue photons (∼450 nm) are more energetic than red photons (∼650 nm). The frequency (ν) and wavelength (λ) of light are related by:
where c is the velocity of light (3.0×10 8 m·s −1 ), and the energy of a particular wavelength (λ) of light is given by:
Thus 1 mol of 680 nm photons of red light has an energy of 176 kJ·mol −1 .
The electrons within the delocalized π system of the pigment have the ability to jump up from the lowest occupied molecular orbital (ground state) to higher unoccupied molecular electron orbitals (excited states) via the absorption of specific wavelengths of light in the visible range (400–725 nm). Chlorophyll has two excited states known as S 1 and S 2 and, upon interaction of the molecule with a photon of light, one of its π electrons is promoted from the ground state (S 0 ) to an excited state, a process taking just 10 −15 s ( Figure 8 ). The energy gap between the S 0 and S 1 states is spanned by the energy provided by a red photon (∼600–700 nm), whereas the energy gap between the S 0 and S 2 states is larger and therefore requires a more energetic (shorter wavelength, higher frequency) blue photon (∼400–500 nm) to span the energy gap.

Photons with slightly different energies (colours) excite each of the vibrational substates of each excited state (as shown by variation in the size and colour of the arrows).
Upon excitation, the electron in the S 2 state quickly undergoes losses of energy as heat through molecular vibration and undergoes conversion into the energy of the S 1 state by a process called internal conversion. Internal conversion occurs on a timescale of 10 −12 s. The energy of a blue photon is thus rapidly degraded to that of a red photon. Excitation of the molecule with a red photon would lead to promotion of an electron to the S 1 state directly. Once the electron resides in the S 1 state, it is lower in energy and thus stable on a somewhat longer timescale (10 −9 s). The energy of the excited electron in the S 1 state can have one of several fates: it could return to the ground state (S 0 ) by emission of the energy as a photon of light (fluorescence), or it could be lost as heat due to internal conversion between S 1 and S 0 . Alternatively, if another chlorophyll is nearby, a process known as excitation energy transfer (EET) can result in the non-radiative exchange of energy between the two molecules ( Figure 9 ). For this to occur, the two chlorophylls must be close by (<7 nm), have a specific orientation with respect to one another, and excited state energies that overlap (are resonant) with one another. If these conditions are met, the energy is exchanged, resulting in a mirror S 0 →S 1 transition in the acceptor molecule and a S 1 →S 0 transition in the other.

Two chlorophyll molecules with resonant S 1 states undergo a mirror transition resulting in the non-radiative transfer of excitation energy between them.
Light-harvesting complexes
In photosynthetic systems, chlorophylls and carotenoids are found attached to membrane-embedded proteins known as light-harvesting complexes (LHCs). Through careful binding and orientation of the pigment molecules, absorbed energy can be transferred among them by EET. Each pigment is bound to the protein by a series of non-covalent bonding interactions (such as, hydrogen bonds, van der Waals interactions, hydrophobic interaction and co-ordination bonds between lone pair electrons of residues such as histidine in the protein and the Mg 2+ ion in chlorophyll); the protein structure is such that each bound pigment experiences a slightly different environment in terms of the surrounding amino acid side chains, lipids, etc., meaning that the S 1 and S 2 energy levels are shifted in energy with respect to that of other neighbouring pigment molecules. The effect is to create a range of pigment energies that act to ‘funnel’ the energy on to the lowest-energy pigments in the LHC by EET.
Reaction centres
A photosystem consists of numerous LHCs that form an antenna of hundreds of pigment molecules. The antenna pigments act to collect and concentrate excitation energy and transfer it towards a ‘special pair’ of chlorophyll molecules that reside in the reaction centre (RC) ( Figure 10 ). Unlike the antenna pigments, the special pair of chlorophylls are ‘redox-active’ in the sense that they can return to the ground state (S 0 ) by the transfer of the electron residing in the S 1 excited state (Chl*) to another species. This process is known as charge separation and result in formation of an oxidized special pair (Chl + ) and a reduced acceptor (A − ). The acceptor in PSII is plastoquinone and in PSI it is ferredoxin. If the RC is to go on functioning, the electron deficiency on the special pair must be made good, in PSII the electron donor is water and in PSI it is plastocyanin.

Light energy is captured by the antenna pigments and transferred to the special pair of RC chlorophylls which undergo a redox reaction leading to reduction of an acceptor molecule. The oxidized special pair is regenerated by an electron donor.
It is worth asking why photosynthetic organisms bother to have a large antenna of pigments serving an RC rather than more numerous RCs. The answer lies in the fact that the special pair of chlorophylls alone have a rather small spatial and spectral cross-section, meaning that there is a limit to the amount of light they can efficiently absorb. The amount of light they can practically absorb is around two orders of magnitude smaller than their maximum possible turnover rate, Thus LHCs act to increase the spatial (hundreds of pigments) and spectral (several types of pigments with different light absorption characteristics) cross-section of the RC special pair ensuring that its turnover rate runs much closer to capacity.
Photosystem II
PSII is a light-driven water–plastoquinone oxidoreductase and is the only enzyme in Nature that is capable of performing the difficult chemistry of splitting water into protons, electrons and oxygen ( Figure 11 ). In principle, water is an extremely poor electron donor since the redox potential of the water–oxygen couple is +820 mV. PSII uses light energy to excite a special pair of chlorophylls, known as P680 due to their 680 nm absorption peak in the red part of the spectrum. P680* undergoes charge separation that results in the formation of an extremely oxidizing species P680 + which has a redox potential of +1200 mV, sufficient to oxidize water. Nonetheless, since water splitting involves four electron chemistry and charge separation only involves transfer of one electron, four separate charge separations (turnovers of PSII) are required to drive formation of one molecule of O 2 from two molecules of water. The initial electron donation to generate the P680 from P680 + is therefore provided by a cluster of manganese ions within the oxygen-evolving complex (OEC), which is attached to the lumen side of PSII ( Figure 12 ). Manganese is a transition metal that can exist in a range of oxidation states from +1 to +5 and thus accumulates the positive charges derived from each light-driven turnover of P680. Progressive extraction of electrons from the manganese cluster is driven by the oxidation of P680 within PSII by light and is known as the S-state cycle ( Figure 12 ). After the fourth turnover of P680, sufficient positive charge is built up in the manganese cluster to permit the splitting of water into electrons, which regenerate the original state of the manganese cluster, protons, which are released into the lumen and contribute to the proton gradient used for ATP synthesis, and the by-product O 2 . Thus charge separation at P680 provides the thermodynamic driving force, whereas the manganese cluster acts as a catalyst for the water-splitting reaction.

The organization of PSII and its light-harvesting antenna. Protein is shown in grey, with chlorophylls in green and carotenoids in orange. Drawn from PDB code 3JCU

Progressive extraction of electrons from the manganese cluster is driven by the oxidation of P680 within PSII by light. Each of the electrons given up by the cluster is eventually repaid at the S 4 to S 0 transition when molecular oxygen (O 2 ) is formed. The protons extracted from water during the process are deposited into the lumen and contribute to the protonmotive force.
The electrons yielded by P680* following charge separation are not passed directly to plastoquinone, but rather via another acceptor called pheophytin, a porphyrin molecule lacking the central magnesium ion as in chlorophyll. Plastoquinone reduction to plastoquinol requires two electrons and thus two molecules of plastoquinol are formed per O 2 molecule evolved by PSII. Two protons are also taken up upon formation of plastoquinol and these are derived from the stroma. PSII is found within the thylakoid membrane of plants as a dimeric RC complex surrounded by a peripheral antenna of six minor monomeric antenna LHC complexes and two to eight trimeric LHC complexes, which together form a PSII–LHCII supercomplex ( Figure 11 ).
Photosystem I
PSI is a light-driven plastocyanin–ferredoxin oxidoreductase ( Figure 13 ). In PSI, the special pair of chlorophylls are known as P700 due to their 700 nm absorption peak in the red part of the spectrum. P700* is an extremely strong reductant that is able to reduce ferredoxin which has a redox potential of −450 mV (and is thus is, in principle, a poor electron acceptor). Reduced ferredoxin is then used to generate NADPH for the Calvin–Benson cycle at a separate complex known as FNR. The electron from P700* is donated via another chlorophyll molecule and a bound quinone to a series of iron–sulfur clusters at the stromal side of the complex, whereupon the electron is donated to ferredoxin. The P700 species is regenerated form P700 + via donation of an electron from the soluble electron carrier protein plastocyanin.

The organization of PSI and its light-harvesting antenna. Protein is shown in grey, with chlorophylls in green and carotenoids in orange. Drawn from PDB code 4XK8.
PSI is found within the thylakoid membrane as a monomeric RC surrounded on one side by four LHC complexes known as LHCI. The PSI–LHCI supercomplex is found mainly in the unstacked regions of the thylakoid membrane ( Figure 13 ).
Other electron transfer chain components
Plastoquinone/plastoquinol.
Plastoquinone is a small lipophilic electron carrier molecule that resides within the thylakoid membrane and carries two electrons and two protons from PSII to the cyt b 6 f complex. It has a very similar structure to that of the molecule ubiquinone (coenzyme Q 10 ) in the mitochondrial inner membrane.
Cytochrome b 6 f complex
The cyt b 6 f complex is a plastoquinol–plastocyanin oxidoreductase and possess a similar structure to that of the cytochrome bc 1 complex (complex III) in mitochondria ( Figure 14 A). As with Complex III, cyt b 6 f exists as a dimer in the membrane and carries out both the oxidation and reduction of quinones via the so-called Q-cycle. The Q-cycle ( Figure 14 B) involves oxidation of one plastoquinol molecule at the Qp site of the complex, both protons from this molecule are deposited in the lumen and contribute to the proton gradient for ATP synthesis. The two electrons, however, have different fates. The first is transferred via an iron–sulfur cluster and a haem cofactor to the soluble electron carrier plastocyanin (see below). The second electron derived from plastoquinol is passed via two separate haem cofactors to another molecule of plastoquinone bound to a separate site (Qn) on the complex, thus reducing it to a semiquinone. When a second plastoquinol molecule is oxidized at Qp, a second molecule of plastocyanin is reduced and two further protons are deposited in the lumen. The second electron reduces the semiquinone at the Qn site which, concomitant with uptake of two protons from the stroma, causes its reduction to plastoquinol. Thus for each pair of plastoquinol molecules oxidized by the complex, one is regenerated, yet all four protons are deposited into the lumen. The Q-cycle thus doubles the number of protons transferred from the stroma to the lumen per plastoquinol molecule oxidized.

( A ) Structure drawn from PDB code 1Q90. ( B ) The protonmotive Q-cycle showing how electrons from plastoquinol are passed to both plastocyanin and plastoquinone, doubling the protons deposited in the lumen for every plastoquinol molecule oxidized by the complex.
Plastocyanin
Plastocyanin is a small soluble electron carrier protein that resides in the thylakoid lumen. The active site of the plastocyanin protein binds a copper ion, which cycles between the Cu 2+ and Cu + oxidation states following its oxidation by PSI and reduction by cyt b 6 f respectively.
Ferredoxin is a small soluble electron carrier protein that resides in the chloroplast stroma. The active site of the ferredoxin protein binds an iron–sulfur cluster, which cycles between the Fe 2+ and Fe 3+ oxidation states following its reduction by PSI and oxidation by the FNR complex respectively.
Ferredoxin–NADP + reductase
The FNR complex is found in both soluble and thylakoid membrane-bound forms. The complex binds a flavin–adenine dinucleotide (FAD) cofactor at its active site, which accepts two electrons from two molecules of ferredoxin before using them reduce NADP + to NADPH.
ATP synthase
The ATP synthase enzyme is responsible for making ATP from ADP and P i ; this endergonic reaction is powered by the energy contained within the protonmotive force. According to the structure, 4.67 H + are required for every ATP molecule synthesized by the chloroplast ATP synthase. The enzyme is a rotary motor which contains two domains: the membrane-spanning F O portion which conducts protons from the lumen to the stroma, and the F 1 catalytic domain that couples this exergonic proton movement to ATP synthesis.
Membrane stacking and the regulation of photosynthesis
Within the thylakoid membrane, PSII–LHCII supercomplexes are packed together into domains known as the grana, which associate with one another to form grana stacks. PSI and ATP synthase are excluded from these stacked PSII–LHCII regions by steric constraints and thus PSII and PSI are segregated in the thylakoid membrane between the stacked and unstacked regions ( Figure 15 ). The cyt b 6 f complex, in contrast, is evenly distributed throughout the grana and stromal lamellae. The evolutionary advantage of membrane stacking is believed to be a higher efficiency of electron transport by preventing the fast energy trap PSI from ‘stealing’ excitation energy from the slower trap PSII, a phenomenon known as spillover. Another possible advantage of membrane stacking in thylakoids may be the segregation of the linear and cyclic electron transfer pathways, which might otherwise compete to reduce plastoquinone. In this view, PSII, cyt b 6 f and a sub-fraction of PSI closest to the grana is involved in linear flow, whereas PSI and cyt b 6 f in the stromal lamellae participates in cyclic flow. The cyclic electron transfer pathway recycles electrons from ferredoxin back to plastoquinone and thus allows protonmotive force generation (and ATP synthesis) without net NADPH production. Cyclic electron transfer thereby provides the additional ATP required for the Calvin–Benson cycle (see below).

( A ) Electron micrograph of the thylakoid membrane showing stacked grana and unstacked stromal lamellae regions. ( B ) Model showing the distribution of the major complexes of photosynthetic electron and proton transfer between the stacked grana and unstacked stromal lamellae regions.
‘Dark’ reactions: the Calvin–Benson cycle
CO 2 is fixed into carbohydrate via the Calvin–Benson cycle in plants, which consumes the ATP and NADPH produced during the light reactions and thus in turn regenerates ADP, P i and NADP + . In the first step of the Calvin–Benson cycle ( Figure 16 ), CO 2 is combined with a 5-carbon (5C) sugar, ribulose 1,5-bisphosphate in a reaction catalysed by the enzyme ribulose-1,5-bisphosphate carboxylase/oxygenase (Rubisco). The reaction forms an unstable 6C intermediate that immediately splits into two molecules of 3-phosphoglycerate. 3-Phosphoglycerate is first phosphorylated by 3-phosphoglycerate kinase using ATP to form 1,3-bisphosphoglycerate. 1,3-Bisphosphoglycerate is then reduced by glyceraldehyde 3-phosphate dehydrogenase using NADPH to form glyceraldehyde 3-phosphate (GAP, a triose or 3C sugar) in reactions, which are the reverse of glycolysis. For every three CO 2 molecules initially combined with ribulose 1,5-bisphopshate, six molecules of GAP are produced by the subsequent steps. However only one of these six molecules can be considered as a product of the Calvin–Benson cycle since the remaining five are required to regenerate ribulose 1,5-bisphosphate in a complex series of reactions that also require ATP. The one molecule of GAP that is produced for each turn of the cycle can be quickly converted by a range of metabolic pathways into amino acids, lipids or sugars such as glucose. Glucose in turn may be stored as the polymer starch as large granules within chloroplasts.

Overview of the biochemical pathway for the fixation of CO 2 into carbohydrate in plants.
A complex biochemical ‘dance’ ( Figure 16 ) is then involved in the regeneration of three ribulose 1,5-bisphosphate (5C) from the remaining five GAP (3C) molecules. The regeneration begins with the conversion of two molecules of GAP into dihydroxyacetone phosphate (DHAP) by triose phosphate isomerase; one of the DHAP molecules is the combined with another GAP molecule to make fructose 1,6-bisphosphate (6C) by aldolase. The fructose 1,6-bisphosphate is then dephosphorylated by fructose-1,6-bisphosphatase to yield fructose 6-phosphate (6C) and releasing P i . Two carbons are then removed from fructose 6-phosphate by transketolase, generating erythrose 4-phosphate (4C); the two carbons are transferred to another molecule of GAP generating xylulose 5-phosphate (5C). Another DHAP molecule, formed from GAP by triose phosphate isomerase is then combined with the erythrose 4-phosphate by aldolase to form sedoheptulose 1,7-bisphosphate (7C). Sedoheptulose 1,7-bisphosphate is then dephosphorylated to sedoheptulose 7-phosphate (7C) by sedoheptulose-1,7-bisphosphatase releasing P i . Sedoheptulose 7-phosphate has two carbons removed by transketolase to produce ribose 5-phosphate (5C) and the two carbons are transferred to another GAP molecule producing another xylulose 5-phosphate (5C). Ribose 5-phosphate and the two molecules of xylulose 5-phosphate (5C) are then converted by phosphopentose isomerase to three molecules of ribulose 5-phosphate (5C). The three ribulose 5-phosphate molecules are then phosphorylated using three ATP by phosphoribulokinase to regenerate three ribulose 1,5-bisphosphate (5C).
Overall the synthesis of 1 mol of GAP requires 9 mol of ATP and 6 mol of NADPH, a required ratio of 1.5 ATP/NADPH. Linear electron transfer is generally thought to supply ATP/NADPH in a ratio of 1.28 (assuming an H + /ATP ratio of 4.67) with the shortfall of ATP believed to be provided by cyclic electron transfer reactions. Since the product of the Calvin cycle is GAP (a 3C sugar) the pathway is often referred to as C 3 photosynthesis and plants that utilize it are called C 3 plants and include many of the world's major crops such as rice, wheat and potato.
Many of the enzymes involved in the Calvin–Benson cycle (e.g. transketolase, glyceraldehyde-3-phosphate dehydrogenase and aldolase) are also involved in the glycolysis pathway of carbohydrate degradation and their activity must therefore be carefully regulated to avoid futile cycling when light is present, i.e. the unwanted degradation of carbohydrate. The regulation of the Calvin–Benson cycle enzymes is achieved by the activity of the light reactions, which modify the environment of the dark reactions (i.e. the stroma). Proton gradient formation across the thylakoid membrane during the light reactions increases the pH and also increases the Mg 2+ concentration in the stroma (as Mg 2+ flows out of the lumen as H + flows in to compensate for the influx of positive charges). In addition, by reducing ferredoxin and NADP + , PSI changes the redox state of the stroma, which is sensed by the regulatory protein thioredoxin. Thioredoxin, pH and Mg 2+ concentration play a key role in regulating the activity of the Calvin–Benson cycle enzymes, ensuring the activity of the light and dark reactions is closely co-ordinated.
It is noteworthy that, despite the complexity of the dark reactions outlined above, the carbon fixation step itself (i.e. the incorporation of CO 2 into carbohydrate) is carried out by a single enzyme, Rubisco. Rubisco is a large multisubunit soluble protein complex found in the chloroplast stroma. The complex consists of eight large (56 kDa) subunits, which contain both catalytic and regulatory domains, and eight small subunits (14 kDa), which enhance the catalytic function of the L subunits ( Figure 17 A). The carboxylation reaction carried out by Rubisco is highly exergonic (Δ G °=−51.9 kJ·mol- 1 ), yet kinetically very slow (just 3 s −1 ) and begins with the protonation of ribulose 1,5-bisphosphate to form an enediolate intermediate which can be combined with CO 2 to form an unstable 6C intermediate that is quickly hydrolysed to yield two 3C 3-phosphoglycerate molecules. The active site in the Rubisco enzyme contains a key lysine residue, which reacts with another (non-substrate) molecule of CO 2 to form a carbamate anion that is then able to bind Mg 2+ . The Mg 2+ in the active site is essential for the catalytic function of Rubisco, playing a key role in binding ribulose 1,5-bisphosphate and activating it such that it readily reacts with CO 2.. Rubisco activity is co-ordinated with that of the light reactions since carbamate formation requires both high Mg 2+ concentration and alkaline conditions, which are provided by the light-driven changes in the stromal environment discussed above ( Figure 17 B).

( A ) Structure of the Rubisco enzyme (the large subunits are shown in blue and the small subunits in green); four of each type of subunit are visible in the image. Drawn from PDB code 1RXO. ( B ) Activation of the lysine residue within the active site of Rubisco occurs via elevated stromal pH and Mg 2+ concentration as a result of the activity of the light reactions.
In addition to carboxylation, Rubisco also catalyses a competitive oxygenation reaction, known as photorespiration, that results in the combination of ribulose 1,5-bisphosphate with O 2 rather than CO 2 . In the oxygenation reaction, one rather than two molecules of 3-phosphoglycerate and one molecule of a 2C sugar known as phosphoglycolate are produced by Rubisco. The phosphoglycolate must be converted in a series of reactions that regenerate one molecule of 3-phosphoglycerate and one molecule of CO 2 . These reactions consume additional ATP and thus result in an energy loss to the plant. Although the oxygenation reaction of Rubisco is much less favourable than the carboxylation reaction, the relatively high concentration of O 2 in the leaf (250 μM) compared with CO 2 (10 μM) means that a significant amount of photorespiration is always occurring. Under normal conditions, the ratio of carboxylation to oxygenation is between 3:1 and 4:1. However, this ratio can be decreased with increasing temperature due to decreased CO 2 concentration in the leaf, a decrease in the affinity of Rubisco for CO 2 compared with O 2 and an increase in the maximum rate of the oxygenation reaction compared with the carboxylation reaction. The inefficiencies of the Rubisco enzyme mean that plants must produce it in very large amounts (∼30–50% of total soluble protein in a spinach leaf) to achieve the maximal photosynthetic rate.
CO 2 -concentrating mechanisms
To counter photorespiration, plants, algae and cyanobacteria have evolved different CO 2 -concentrating mechanisms CCMs that aim to increase the concentration of CO 2 relative to O 2 in the vicinity of Rubisco. One such CCM is C 4 photosynthesis that is found in plants such as maize, sugar cane and savanna grasses. C 4 plants show a specialized leaf anatomy: Kranz anatomy ( Figure 18 ). Kranz, German for wreath, refers to a bundle sheath of cells that surrounds the central vein within the leaf, which in turn are surrounded by the mesophyll cells. The mesophyll cells in such leaves are rich in the enzyme phosphoenolpyruvate (PEP) carboxylase, which fixes CO 2 into a 4C carboxylic acid: oxaloaceatate. The oxaloacetate formed by the mesophyll cells is reduced using NADPH to malate, another 4C acid: malate. The malate is then exported from the mesophyll cells to the bundle sheath cells, where it is decarboxylated to pyruvate thus regenerating NADPH and CO 2 . The CO 2 is then utilized by Rubisco in the Calvin cycle. The pyruvate is in turn returned to the mesophyll cells where it is phosphorylated using ATP to reform PEP ( Figure 19 ). The advantage of C 4 photosynthesis is that CO 2 accumulates at a very high concentration in the bundle sheath cells that is then sufficient to allow Rubisco to operate efficiently.

Plants growing in hot, bright and dry conditions inevitably have to have their stomata closed for large parts of the day to avoid excessive water loss and wilting. The net result is that the internal CO 2 concentration in the leaf is very low, meaning that C 3 photosynthesis is not possible. To counter this limitation, another CCM is found in succulent plants such as cacti. The Crassulaceae fix CO 2 into malate during the day via PEP carboxylase, store it within the vacuole of the plant cell at night and then release it within their tissues by day to be fixed via normal C 3 photosynthesis. This is termed crassulacean acid metabolism (CAM).
Acknowledgments
I thank Professor Colin Osborne (University of Sheffield, Sheffield, U.K.) for useful discussions on the article, Dr Dan Canniffe (Penn State University, Pennsylvania, PA, U.S.A.) for providing pure pigment spectra and Dr P.J. Weaire (Kingston University, Kingston-upon-Thames, U.K.) for his original Photosynthesis BASC article (1994) on which this essay is partly based.
Abbreviations
This article is a reviewed, revised and updated version of the following ‘Biochemistry Across the School Curriculum’ (BASC) booklet: Weaire, P.J. (1994) Photosynthesis . For further information and to provide feedback on this or any other Biochemical Society education resource, please contact [email protected]. For further information on other Biochemical Society publications, please visit www.biochemistry.org/publications .
Competing Interests
The Author declares that there are no competing interests associated with this article.
Recommended reading and key publications
- Blankenship R.E. Early evolution of photosynthesis. Plant Physiol. 2010; 154 :434–438. doi: 10.1104/pp.110.161687. [ PMC free article ] [ PubMed ] [ CrossRef ] [ Google Scholar ]
- Blankenship R.E. Molecular Mechanisms of Photosynthesis. Chichester: Wiley–Blackwell Publishing; 2014. [ Google Scholar ]
- Nelson N., Ben Shem A. The complex architecture of oxygenic photosynthesis. Nat. Rev. 2004; 5 :1–12. doi: 10.1038/nrm1525. [ PubMed ] [ CrossRef ] [ Google Scholar ]
- Raines C. The Calvin cycle revisited. Photosynth. Res. 2003; 75 :1–10. doi: 10.1023/A:1022421515027. [ PubMed ] [ CrossRef ] [ Google Scholar ]
- Ruban A.V. Evolution under the sun: optimizing light harvesting in photosynthesis. J. Exp. Bot. 2015; 66 :7–23. doi: 10.1093/jxb/eru400. [ PubMed ] [ CrossRef ] [ Google Scholar ]
- Sage R.F. The evolution of C 4 photosynthesis. New Phytol. 2004; 161 :341–370. doi: 10.1111/j.1469-8137.2004.00974.x. [ PubMed ] [ CrossRef ] [ Google Scholar ]

IMAGES
VIDEO
COMMENTS
Photosynthesis is critical for the existence of the vast majority of life on Earth. It is the way in which virtually all energy in the biosphere becomes available to living things. As primary producers, photosynthetic organisms form the base of Earth's food webs and are consumed directly or indirectly by all higher life-forms. Additionally ...
Photosynthesis is important to living organisms because it is the number one source of oxygen in the atmosphere. Without photosynthesis, the carbon cycle could not occur, oxygen-requiring life would not survive and plants would die. Green plants and trees use photosynthesis to make food from sunlight, carbon dioxide and water in the atmosphere ...
Most life on Earth depends on photosynthesis.The process is carried out by plants, algae, and some types of bacteria, which capture energy from sunlight to produce oxygen (O 2) and chemical energy stored in glucose (a sugar). Herbivores then obtain this energy by eating plants, and carnivores obtain it by eating herbivores.. The process. During photosynthesis, plants take in carbon dioxide (CO ...
Photosynthesis is essential to all life on earth. It is the only biological process that captures energy from outer space (sunlight) and converts it into chemical energy in the form of G3P ... The importance of photosynthesis is not just that it can capture sunlight's energy. A lizard sunning itself on a cold day can use the sun's energy to ...
During photosynthesis in green plants, light energy is captured and used to convert water, carbon dioxide, and minerals into oxygen and energy-rich organic compounds. It would be impossible to overestimate the importance of photosynthesis in the maintenance of life on Earth. If photosynthesis ceased, there would soon be little food or other ...
Essays Biochem (2016) 60 (3): 255-273. Photosynthesis sustains virtually all life on planet Earth providing the oxygen we breathe and the food we eat; it forms the basis of global food chains and meets the majority of humankind's current energy needs through fossilized photosynthetic fuels.
Photosynthesis is carried out by photosynthetic organisms. Photosynthesis drives the movement of matter, or atoms, between organisms and the environment. Photosynthetic organisms take in and use carbon dioxide and water from the air and soil. Photosynthetic organisms release oxygen into the air. Organisms throughout the ecosystem use this ...
Photosynthesis ( / ˌfoʊtəˈsɪnθəsɪs / FOH-tə-SINTH-ə-sis) [1] is a system of biological processes by which photosynthetic organisms, such as most plants, algae, and cyanobacteria, convert light energy, typically from sunlight, into the chemical energy necessary to fuel their activities.
Photosynthesis. Photosynthesis is the processes of using sunlight to convert chemical compounds (specifically carbon dioxide and water) into food. Photosynthesizing organisms (plants, algae, and bacteria) provide most of the chemical energy that flows through the biosphere. They also produced most of the biomass that led to the fossil fuels ...
Why is photosynthesis important to animals? Photosynthesis offers similar benefits to animals as it does to humans. Again, photosynthesis provides the oxygen needed to support animal life.
Figure 8.1.1 8.1. 1: This world map shows Earth's distribution of photosynthesis as seen via chlorophyll a concentrations. On land, this is evident via terrestrial plants, and in oceanic zones, via phytoplankton. (credit: modification of work by SeaWiFS Project, NASA/Goddard Space Flight Center and ORBIMAGE) The processes in all organisms ...
Photosynthesis is essential for the existence of all life on earth. It serves a crucial role in the food chain - the plants create their food using this process, thereby, forming the primary producers. Photosynthesis is also responsible for the production of oxygen - which is needed by most organisms for their survival.
Photosynthesis is a chemical process whereby plants and algae that contain chlorophyll capture radiant energy from the sun, and use carbon dioxide and water from the environment to then convert the sunlight to food (glucose), while at the same time creating oxygen and water as byproducts. Of course, we humans get the benefit of all that food ...
Introduction. Photosynthesis is the chemical reaction that sustains most life on Earth. Since the description of the Hill reaction and the Calvin-Benson cycle 1-3, knowledge about their components, regulation, and limitations experienced a vertiginous increase.It is widely known that plants have important handicaps related to photosynthesis.
Photosynthesis is the process in which green plants use sunlight to make their own food. Photosynthesis is necessary for life on Earth. Without it there would be no green plants, and without green plants there would be no animals. Interactive
Photosynthesis is very important for life on Earth. It gives us oxygen, which we need to breathe. Plants use the glucose they make for growth and to build other important substances like cellulose, which they use to make their cell walls. Without photosynthesis, there would be no food for animals or people, and no oxygen to breathe.
Photo Credits. Photosynthesis is the conversion of light energy into chemical energy. It is the primary process for life on Earth. Almost all living things on the planet rely on photosynthesis to provide their energy, either directly or indirectly. The importance of photosynthesis to man cannot be understated.
It is the main event in light reactions of photosynthesis. The function of light reactions is two fold —. (1) The photochemical splitting of water provides hydrogen atoms for the reduction of CO 2, and. (2) Producing of ATP which provides energy for the subsequent synthesis of carbohydrates.
The plants requires glucose for their growth and energy. All the living people and animals on the earth depend on fats, proteins, and carbohydrates to obtain their energy, that is what makes this is one of the reasons photosynthesis is essential to life on earth. Another product of photosynthesis is oxygen which we need to breathe.
The Sun provides energy to the Earth in the form of radiant (light) energy and heat energy. Photosynthesis is the process whereby green plants use carbon dioxide from the air, water from the soil and radiant energy from the Sun in a series of chemical reactions to produce glucose (food) and oxygen.
Nearly 240 years after Joseph Priestley's influential experiments involving a mouse, a plant and a bell jar the need and desire to study photosynthesis and the environment has not diminished. In fact, it is well recognized that the relationship between photosynthesis and the environment is key to understanding the health of our planet, in ...
It is the biochemical process that sustains the biosphere as the basis for the food chain. The oxygen produced as a by-product of photosynthesis allowed the formation of the ozone layer, the evolution of aerobic respiration and thus complex multicellular life. Oxygenic photosynthesis involves the conversion of water and CO 2 into complex ...
Without photosynthesis plant life would be unable to create its own food and sustain themselves causing them to die. Earth would lack the oxygen and energy that plant life provides. ... Importance Of Plants Essay 2023 Words | 9 Pages. Introduction Plants are a major necessity in the balance of nature, people's lives, and our terrain. We may ...
4.2 (81 reviews) Which is an example of why the process of photosynthesis is important to life on Earth? A) Grass uses photosynthesis to produce glucose, which is used within the grass for growth. B) Fungi use photosynthesis to decompose dead and decaying plant matter. C) Cold-blooded animals use photosynthesis to convert sunlight into energy ...