Thank you for visiting nature.com. You are using a browser version with limited support for CSS. To obtain the best experience, we recommend you use a more up to date browser (or turn off compatibility mode in Internet Explorer). In the meantime, to ensure continued support, we are displaying the site without styles and JavaScript.
- View all journals
- Explore content
- About the journal
- Publish with us
- Sign up for alerts
- Review Article
- Published: 06 February 2013

Malaria biology and disease pathogenesis: insights for new treatments
- Louis H Miller 1 ,
- Hans C Ackerman 1 ,
- Xin-zhuan Su 1 &
- Thomas E Wellems 1
Nature Medicine volume 19 , pages 156–167 ( 2013 ) Cite this article
26k Accesses
408 Citations
9 Altmetric
Metrics details
- Pathogenesis
- Therapeutics
Plasmodium falciparum malaria, an infectious disease caused by a parasitic protozoan, claims the lives of nearly a million children each year in Africa alone and is a top public health concern. Evidence is accumulating that resistance to artemisinin derivatives, the frontline therapy for the asexual blood stage of the infection, is developing in southeast Asia. Renewed initiatives to eliminate malaria will benefit from an expanded repertoire of antimalarials, including new drugs that kill circulating P. falciparum gametocytes, thereby preventing transmission. Our current understanding of the biology of asexual blood-stage parasites and gametocytes and the ability to culture them in vitro lends optimism that high-throughput screenings of large chemical libraries will produce a new generation of antimalarial drugs. There is also a need for new therapies to reduce the high mortality of severe malaria. An understanding of the pathophysiology of severe disease may identify rational targets for drugs that improve survival.
This is a preview of subscription content, access via your institution
Access options
Subscribe to this journal
Receive 12 print issues and online access
195,33 € per year
only 16,28 € per issue
Buy this article
- Purchase on Springer Link
- Instant access to full article PDF
Prices may be subject to local taxes which are calculated during checkout
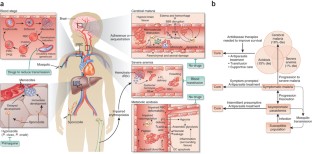
Similar content being viewed by others
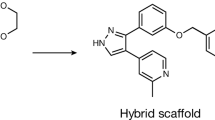
A Gram-negative-selective antibiotic that spares the gut microbiome
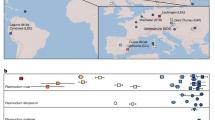
Ancient Plasmodium genomes shed light on the history of human malaria
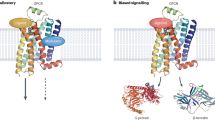
Know your molecule: pharmacological characterization of drug candidates to enhance efficacy and reduce late-stage attrition
Delves, M. et al. The activities of current antimalarial drugs on the life cycle stages of Plasmodium : a comparative study with human and rodent parasites. PLoS Med. 9 , e1001169 (2012).
PubMed PubMed Central Google Scholar
Wells, T.N., Burrows, J.N. & Baird, J.K. Targeting the hypnozoite reservoir of Plasmodium vivax : the hidden obstacle to malaria elimination. Trends Parasitol. 26 , 145–151 (2010).
Article PubMed Google Scholar
Burrows, J.N., Chibale, K. & Wells, T.N. The state of the art in anti-malarial drug discovery and development. Curr. Top. Med. Chem. 11 , 1226–1254 (2011).
CAS PubMed Google Scholar
Drew, M.E. et al. Plasmodium food vacuole plasmepsins are activated by falcipains. J. Biol. Chem. 283 , 12870–12876 (2008).
CAS PubMed PubMed Central Google Scholar
Fitch, C.D. et al. Lysis of Plasmodium falciparum by ferriprotoporphyrin IX and a chloroquine-ferriprotoporphyrin IX complex. Antimicrob. Agents Chemother. 21 , 819–822 (1982).
Lehane, A.M., McDevitt, C.A., Kirk, K. & Fidock, D.A. Degrees of chloroquine resistance in Plasmodium —is the redox system involved? Int. J. Parasitol. Drugs Drug Resist. 2 , 47–57 (2012).
PubMed Google Scholar
Fidock, D.A. et al. Mutations in the P. falciparum digestive vacuole transmembrane protein PfCRT and evidence for their role in chloroquine resistance. Mol. Cell 6 , 861–871 (2000).
Martin, R.E. et al. Chloroquine transport via the malaria parasite's chloroquine resistance transporter. Science 325 , 1680–1682 (2009).
Cowman, A.F., Karcz, S., Galatis, D. & Culvenor, J.G. A P-glycoprotein homologue of Plasmodium falciparum is localized on the digestive vacuole. J. Cell Biol. 113 , 1033–1042 (1991).
Mu, J. et al. Multiple transporters associated with malaria parasite responses to chloroquine and quinine. Mol. Microbiol. 49 , 977–989 (2003).
van Schalkwyk, D.A. & Egan, T.J. Quinoline-resistance reversing agents for the malaria parasite Plasmodium falciparum . Drug Resist. Updat. 9 , 211–226 (2006).
Cooper, R.A. et al. Mutations in transmembrane domains 1, 4 and 9 of the Plasmodium falciparum chloroquine resistance transporter alter susceptibility to chloroquine, quinine and quinidine. Mol. Microbiol. 63 , 270–282 (2007).
Sá, J.M. et al. Geographic patterns of Plasmodium falciparum drug resistance distinguished by differential responses to amodiaquine and chloroquine. Proc. Natl. Acad. Sci. USA 106 , 18883–18889 (2009).
De, D., Krogstad, F.M., Cogswell, F.B. & Krogstad, D.J. Aminoquinolines that circumvent resistance in Plasmodium falciparum in vitro . Am. J. Trop. Med. Hyg. 55 , 579–583 (1996).
Hawley, S.R. et al. Manipulation of the N-alkyl substituent in amodiaquine to overcome the verapamil-sensitive chloroquine resistance component. Antimicrob. Agents Chemother. 40 , 2345–2349 (1996).
Madrid, P.B., Liou, A.P., DeRisi, J.L. & Guy, R.K. Incorporation of an intramolecular hydrogen-bonding motif in the side chain of 4-aminoquinolines enhances activity against drug-resistant P. falciparum . J. Med. Chem. 49 , 4535–4543 (2006).
Hwang, J.Y. et al. Synthesis and evaluation of 7-substituted 4-aminoquinoline analogues for antimalarial activity. J. Med. Chem. 54 , 7084–7093 (2011).
Pou, S. et al. Sontochin as a guide to the development of drugs against chloroquine-resistant malaria. Antimicrob. Agents Chemother. 56 , 3475–3480 (2012).
O'Neill, P.M. et al. Candidate selection and preclinical evaluation of N-tert-butyl isoquine (GSK369796), an affordable and effective 4-aminoquinoline antimalarial for the 21 st century. J. Med. Chem. 52 , 1408–1415 (2009).
Sowunmi, A. et al. Predictors of the failure of treatment with chloroquine plus chlorpheniramine, in children with acute, uncomplicated, Plasmodium falciparum malaria. Ann. Trop. Med. Parasitol. 99 , 331–338 (2005).
Zishiri, V.K. et al. Quinoline antimalarials containing a dibemethin group are active against chloroquinone-resistant Plasmodium falciparum and inhibit chloroquine transport via the P. falciparum chloroquine-resistance transporter (PfCRT). J. Med. Chem. 54 , 6956–6968 (2011).
Burgess, S.J. et al. A chloroquine-like molecule designed to reverse resistance in Plasmodium falciparum . J. Med. Chem. 49 , 5623–5625 (2006).
Dive, D. & Biot, C. Ferrocene conjugates of chloroquine and other antimalarials: the development of ferroquine, a new antimalarial. ChemMedChem 3 , 383–391 (2008).
Mombo-Ngoma, G. et al. Phase I randomized dose-ascending placebo-controlled trials of ferroquine–a candidate anti-malarial drug–in adults with asymptomatic Plasmodium falciparum infection. Malar. J. 10 , 53 (2011).
Mzayek, F. et al. Randomized dose-ranging controlled trial of AQ-13, a candidate antimalarial, and chloroquine in healthy volunteers. PLoS Clin. Trials 2 , e6 (2007).
Goldberg, D.E. et al. Probing the chloroquine resistance locus of Plasmodium falciparum with a novel class of multidentate metal(III) coordination complexes. J. Biol. Chem. 272 , 6567–6572 (1997).
Yuan, J. et al. Chemical genomic profiling for antimalarial therapies, response signatures, and molecular targets. Science 333 , 724–729 (2011).
Michel, J.B., Yeh, P.J., Chait, R., Moellering, R.C. Jr. & Kishony, R. Drug interactions modulate the potential for evolution of resistance. Proc. Natl. Acad. Sci. USA 105 , 14918–14923 (2008).
Chait, R., Craney, A. & Kishony, R. Antibiotic interactions that select against resistance. Nature 446 , 668–671 (2007).
Klonis, N. et al. Artemisinin activity against Plasmodium falciparum requires hemoglobin uptake and digestion. Proc. Natl. Acad. Sci. USA 108 , 11405–11410 (2011).
Hartwig, C.L. et al. Accumulation of artemisinin trioxane derivatives within neutral lipids of Plasmodium falciparum malaria parasites is endoperoxide-dependent. Biochem. Pharmacol. 77 , 322–336 (2009).
Kannan, R., Sahal, D. & Chauhan, V.S. Heme-artemisinin adducts are crucial mediators of the ability of artemisinin to inhibit heme polymerization. Chem. Biol. 9 , 321–332 (2002).
White, N.J. Qinghaosu (artemisinin): the price of success. Science 320 , 330–334 (2008).
Baird, J.K. Real-world therapies and the problem of vivax malaria. N. Engl. J. Med. 359 , 2601–2603 (2008).
Dondorp, A.M. et al. Artesunate versus quinine in the treatment of severe falciparum malaria in African children (AQUAMAT): an open-label, randomised trial. Lancet 376 , 1647–1657 (2010).
Li, G.Q., Guo, X.B., Fu, L.C., Jian, H.X. & Wang, X.H. Clinical trials of artemisinin and its derivatives in the treatment of malaria in China. Trans. R. Soc. Trop. Med. Hyg. 88 (suppl. 1), S5–S6 (1994).
Noedl, H. et al. Evidence of artemisinin-resistant malaria in western Cambodia. N. Engl. J. Med. 359 , 2619–2620 (2008).
Dondorp, A.M. et al. Artemisinin resistance in Plasmodium falciparum malaria. N. Engl. J. Med. 361 , 455–467 (2009).
Dondorp, A.M. et al. The threat of artemisinin-resistant malaria. N. Engl. J. Med. 365 , 1073–1075 (2011).
Noedl, H., Socheat, D. & Satimai, W. Artemisinin-resistant malaria in Asia. N. Engl. J. Med. 361 , 540–541 (2009).
Noedl, H. et al. Artemisinin resistance in Cambodia: a clinical trial designed to address an emerging problem in Southeast Asia. Clin. Infect. Dis. 51 , e82–e89 (2010).
Anderson, T.J. et al. High heritability of malaria parasite clearance rate indicates a genetic basis for artemisinin resistance in western Cambodia. J. Infect. Dis. 201 , 1326–1330 (2010).
Mok, S. et al. Artemisinin resistance in Plasmodium falciparum is associated with an altered temporal pattern of transcription. BMC Genomics 12 , 391 (2011).
Cheeseman, I.H. et al. A major genome region underlying artemisinin resistance in malaria. Science 336 , 79–82 (2012).
O'Neill, P.M. & Posner, G.H. A medicinal chemistry perspective on artemisinin and related endoperoxides. J. Med. Chem. 47 , 2945–2964 (2004).
Jefford, C.W. Synthetic peroxides as potent antimalarials. News and views. Curr. Top. Med. Chem. 12 , 373–399 (2012).
Vennerstrom, J.L. et al. Identification of an antimalarial synthetic trioxolane drug development candidate. Nature 430 , 900–904 (2004).
Charman, S.A. et al. Synthetic ozonide drug candidate OZ439 offers new hope for a single-dose cure of uncomplicated malaria. Proc. Natl. Acad. Sci. USA 108 , 4400–4405 (2011).
Doerig, C. et al. Malaria: targeting parasite and host cell kinomes. Biochim. Biophys. Acta 1804 , 604–612 (2010).
Doerig, C. et al. Protein kinases of malaria parasites: an update. Trends Parasitol. 24 , 570–577 (2008).
Kato, N. et al. Gene expression signatures and small-molecule compounds link a protein kinase to Plasmodium falciparum motility. Nat. Chem. Biol. 4 , 347–356 (2008).
Lemercier, G. et al. Identification and characterization of novel small molecules as potent inhibitors of the plasmodial calcium-dependent protein kinase 1. Biochemistry 48 , 6379–6389 (2009).
Le Roch, K. et al. Activation of a Plasmodium falciparum cdc2-related kinase by heterologous p25 and cyclin H. Functional characterization of a P. falciparum cyclin homologue. J. Biol. Chem. 275 , 8952–8958 (2000).
Xiao, Z., Waters, N.C., Woodard, C.L., Li, Z. & Li, P.K. Design and synthesis of Pfmrk inhibitors as potential antimalarial agents. Bioorg. Med. Chem. Lett. 11 , 2875–2878 (2001).
Bouloc, N. et al. Synthesis and in vitro evaluation of imidazopyridazines as novel inhibitors of the malarial kinase PfPK7. Bioorg. Med. Chem. Lett. 18 , 5294–5298 (2008).
Desoubzdanne, D. et al. Alisiaquinones and alisiaquinol, dual inhibitors of Plasmodium falciparum enzyme targets from a New Caledonian deep water sponge. J. Nat. Prod. 71 , 1189–1192 (2008).
Laurent, D. et al. Antimalarial potential of xestoquinone, a protein kinase inhibitor isolated from a Vanuatu marine sponge Xestospongia sp. Bioorg. Med. Chem. 14 , 4477–4482 (2006).
McRobert, L. et al. Gametogenesis in malaria parasites is mediated by the cGMP-dependent protein kinase. PLoS Biol. 6 , e139 (2008).
Hayton, K. et al. Erythrocyte binding protein PfRH5 polymorphisms determine species-specific pathways of Plasmodium falciparum invasion. Cell Host Microbe 4 , 40–51 (2008).
Baum, J. et al. Reticulocyte-binding protein homologue 5—an essential adhesin involved in invasion of human erythrocytes by Plasmodium falciparum . Int. J. Parasitol. 39 , 371–380 (2009).
Chen, L. et al. An EGF-like protein forms a complex with PfRh5 and is required for invasion of human erythrocytes by Plasmodium falciparum . PLoS Pathog. 7 , e1002199 (2011).
Crosnier, C. et al. Basigin is a receptor essential for erythrocyte invasion by Plasmodium falciparum . Nature 480 , 534–537 (2011).
Pizarro, J.C. et al. Crystal structure of the malaria vaccine candidate apical membrane antigen 1. Science 308 , 408–411 (2005).
Tyler, J.S. & Boothroyd, J.C. The C-terminus of toxoplasma RON2 provides the crucial link between AMA1 and the host-associated invasion complex. PLoS Pathog. 7 , e1001282 (2011).
Lamarque, M. et al. The RON2–AMA1 interaction is a critical step in moving junction-dependent invasion by apicomplexan parasites. PLoS Pathog. 7 , e1001276 (2011).
Srinivasan, P. et al. Binding of Plasmodium merozoite proteins RON2 and AMA1 triggers commitment to invasion. Proc. Natl. Acad. Sci. USA 108 , 13275–13280 (2011).
Gao, M. & Skolnick, J. The distribution of ligand-binding pockets around protein-protein interfaces suggests a general mechanism for pocket formation. Proc. Natl. Acad. Sci. USA 109 , 3784–3789 (2012).
Singh, S., Alam, M.M., Pal-Bhowmick, I., Brzostowski, J.A. & Chitnis, C.E. Distinct external signals trigger sequential release of apical organelles during erythrocyte invasion by malaria parasites. PLoS Pathog. 6 , e1000746 (2010).
Leykauf, K. et al. Protein kinase a dependent phosphorylation of apical membrane antigen 1 plays an important role in erythrocyte invasion by the malaria parasite. PLoS Pathog. 6 , e1000941 (2010).
Aikawa, M., Miller, L.H., Johnson, J. & Rabbege, J. Erythrocyte entry by malarial parasites. A moving junction between erythrocyte and parasite. J. Cell Biol. 77 , 72–82 (1978).
Riglar, D.T. et al. Super-resolution dissection of coordinated events during malaria parasite invasion of the human erythrocyte. Cell Host Microbe 9 , 9–20 (2011).
Dvorin, J.D. et al. A plant-like kinase in Plasmodium falciparum regulates parasite egress from erythrocytes. Science 328 , 910–912 (2010).
Kafsack, B.F.C. et al. Rapid membrane disruption by a perforin-like protein facilitates parasite exit from host cells. Science 323 , 530–533 (2009).
Marsh, K. et al. Indicators of life-threatening malaria in African children. N. Engl. J. Med. 332 , 1399–1404 (1995).
Planche, T. et al. Assessment of volume depletion in children with malaria. PLoS Med. 1 , e18 (2004).
Maitland, K. et al. Mortality after fluid bolus in African children with severe infection. N. Engl. J. Med. 364 , 2483–2495 (2011).
Beare, N.A. et al. Prognostic significance and course of retinopathy in children with severe malaria. Arch. Ophthalmol. 122 , 1141–1147 (2004).
Taylor, T.E. et al. Differentiating the pathologies of cerebral malaria by postmortem parasite counts. Nat. Med. 10 , 143–145 (2004).
Dondorp, A.M. et al. Direct in vivo assessment of microcirculatory dysfunction in severe falciparum malaria. J. Infect. Dis. 197 , 79–84 (2008).
Francischetti, I.M.B. et al. Plasmodium falciparum -infected erythrocytes induce tissue factor expression in endothelial cells and support the assembly of multimolecular coagulation complexes. J. Thromb. Haemost. 5 , 155–165 (2007).
Taoufiq, Z. et al. Rho kinase inhibition in severe malaria: thwarting parasite-induced collateral damage to endothelia. J. Infect. Dis. 197 , 1062–1073 (2008).
Cabrales, P., Zanini, G.M., Meays, D., Frangos, J.A. & Carvalho, L.J.M. Murine cerebral malaria is associated with a vasospasm-like microcirculatory dysfunction, and survival upon rescue treatment is markedly increased by nimodipine. Am. J. Pathol. 176 , 1306–1315 (2010).
Yeo, T.W. et al. Impaired nitric oxide bioavailability and l-arginine reversible endothelial dysfunction in adults with falciparum malaria. J. Exp. Med. 204 , 2693–2704 (2007).
Lavstsen, T. et al. Plasmodium falciparum erythrocyte membrane protein 1 domain cassettes 8 and 13 are associated with severe malaria in children. Proc. Natl. Acad. Sci. USA 109 , E1791–E1800 (2012).
Claessens, A. et al. A subset of group A-like var genes encodes the malaria parasite ligands for binding to human brain endothelial cells. Proc. Natl. Acad. Sci. USA 109 , E1772–E1781 (2012).
Avril, M. et al. A restricted subset of var genes mediates adherence of Plasmodium falciparum -infected erythrocytes to brain endothelial cells. Proc. Natl. Acad. Sci. USA 109 , E1782–E1790 (2012).
Vouret-Craviari, V. & Grall, D. Van Obberghen-Schilling, E. Modulation of Rho GTPase activity in endothelial cells by selective proteinase-activated receptor (PAR) agonists. J. Thromb. Haemost. 1 , 1103–1111 (2003).
Hemmer, C.J., Kern, P., Holst, F.G., Nawroth, P.P. & Dietrich, M. Neither heparin nor acetylsalicylic acid influence the clinical course in human Plasmodium falciparum malaria: a prospective randomized study. Am. J. Trop. Med. Hyg. 45 , 608–612 (1991).
Vogetseder, A., Ospelt, C., Reindl, M., Schober, M. & Schmutzhard, E. Time course of coagulation parameters, cytokines and adhesion molecules in Plasmodium falciparum malaria. Trop. Med. Int. Health 9 , 767–773 (2004).
Francischetti, I.M.B. et al. Defibrotide interferes with several steps of the coagulation-inflammation cycle and exhibits therapeutic potential to treat severe malaria. Arterioscler. Thromb. Vasc. Biol. 32 , 786–798 (2012).
de Mast, Q. et al. ADAMTS13 deficiency with elevated levels of ultra-large and active von Willebrand factor in P. falciparum and P. vivax malaria. Am. J. Trop. Med. Hyg. 80 , 492–498 (2009).
Bridges, D.J. et al. Rapid activation of endothelial cells enables Plasmodium falciparum adhesion to platelet-decorated von Willebrand factor strings. Blood 115 , 1472–1474 (2010).
Matsushita, K. et al. Nitric oxide regulates exocytosis by S-nitrosylation of N-ethylmaleimide–sensitive factor. Cell 115 , 139–150 (2003).
Yeo, T.W. et al. Angiopoietin-2 is associated with decreased endothelial nitric oxide and poor clinical outcome in severe falciparum malaria. Proc. Natl. Acad. Sci. USA 105 , 17097–17102 (2008).
Conroy, A.L. et al. Angiopoietin-2 levels are associated with retinopathy and predict mortality in Malawian children with cerebral malaria: a retrospective case-control study. Crit. Care Med. 40 , 952–959 (2012).
Casals-Pascual, C. et al. High levels of erythropoietin are associated with protection against neurological sequelae in African children with cerebral malaria. Proc. Natl. Acad. Sci. USA 105 , 2634–2639 (2008).
Kaiser, K. et al. Recombinant human erythropoietin prevents the death of mice during cerebral malaria. J. Infect. Dis. 193 , 987–995 (2006).
Yeo, T.W. et al. Increased asymmetric dimethylarginine in severe falciparum malaria: association with impaired nitric oxide bioavailability and fatal outcome. PLoS Pathog. 6 , e1000868 (2010).
Janka, J.J. et al. Increased pulmonary pressures and myocardial wall stress in children with severe malaria. J. Infect. Dis. 202 , 791–800 (2010).
Walther, M. et al. HMOX1 gene promoter alleles and high HO-1 levels are associated with severe malaria in Gambian children. PLoS Pathog. 8 , e1002579 (2012).
Omodeo-Sale, F., Cortelezzi, L., Vommaro, Z., Scaccabarozzi, D. & Dondorp, A. Dysregulation of l-arginine metabolism and bioavailability associated to free plasma heme. Am. J. Physiol. Cell Physiol. 299 , C148–C154 (2010).
Kato, G.J. et al. Endogenous nitric oxide synthase inhibitors in sickle cell disease: abnormal levels and correlations with pulmonary hypertension, desaturation, haemolysis, organ dysfunction and death. Br. J. Haematol. 145 , 506–513 (2009).
Davids, M. et al. Role of the human erythrocyte in generation and storage of asymmetric dimethylarginine. Am. J. Physiol. Heart Circ. Physiol. 302 , H1762–H1770 (2012).
Wojciak-Stothard, B. et al. The ADMA/DDAH pathway is a critical regulator of endothelial cell motility. J. Cell Sci. 120 , 929–942 (2007).
Leiper, J. et al. Disruption of methylarginine metabolism impairs vascular homeostasis. Nat. Med. 13 , 198–203 (2007).
Jallow, M. et al. Genome-wide and fine-resolution association analysis of malaria in West Africa. Nat. Genet. 41 , 657–665 (2009).
Reiter, C.D. et al. Cell-free hemoglobin limits nitric oxide bioavailability in sickle-cell disease. Nat. Med. 8 , 1383–1389 (2002).
Yeo, T.W. et al. Relationship of cell-free hemoglobin to impaired endothelial nitric oxide bioavailability and perfusion in severe falciparum malaria. J. Infect. Dis. 200 , 1522–1529 (2009).
Huie, R.E. & Padmaja, S. The reaction of NO with superoxide. Free Radic. Res. Commun. 18 , 195–199 (1993).
De Caterina, R. et al. Nitric oxide decreases cytokine-induced endothelial activation. Nitric oxide selectively reduces endothelial expression of adhesion molecules and proinflammatory cytokines. J. Clin. Invest. 96 , 60–68 (1995).
Yang, Y. & Loscalzo, J. Regulation of tissue factor expression in human microvascular endothelial cells by nitric oxide. Circulation 101 , 2144–2148 (2000).
Loscalzo, J. Nitric oxide insufficiency, platelet activation, and arterial thrombosis. Circ. Res. 88 , 756–762 (2001).
Serirom, S. et al. Anti-adhesive effect of nitric oxide on Plasmodium falciparum cytoadherence under flow. Am. J. Pathol. 162 , 1651–1660 (2003).
Cosby, K. et al. Nitrite reduction to nitric oxide by deoxyhemoglobin vasodilates the human circulation. Nat. Med. 9 , 1498–1505 (2003).
Huang, Z. et al. Enzymatic function of hemoglobin as a nitrite reductase that produces NO under allosteric control. J. Clin. Invest. 115 , 2099–2107 (2005).
Gladwin, M.T. & Kim-Shapiro, D. The functional nitrite reductase activity of the heme-globins. Blood 112 , 2636–2647 (2008).
Li, H., Cui, H., Kundu, T.K., Alzawahra, W. & Zweier, J.L. Nitric oxide production from nitrite occurs primarily in tissues not in the blood: critical role of xanthine oxidase and aldehyde oxidase. J. Biol. Chem. 283 , 17855–17863 (2008).
Minneci, P.C. et al. Nitrite reductase activity of hemoglobin as a systemic nitric oxide generator mechanism to detoxify plasma hemoglobin produced during hemolysis. Am. J. Physiol. Heart Circ. Physiol. 295 , H743–H754 (2008).
Yu, B. et al. Inhaled nitric oxide enables artificial blood transfusion without hypertension. Circulation 117 , 1982–1990 (2008).
Duranski, M.R. et al. Cytoprotective effects of nitrite during in vivo ischemia-reperfusion of the heart and liver. J. Clin. Invest. 115 , 1232–1240 (2005).
Cauwels, A. et al. Nitrite protects against morbidity and mortality associated with TNF- or LPS-induced shock in a soluble guanylate cyclase–dependent manner. J. Exp. Med. 206 , 2915–2924 (2009).
Erez, A. et al. Requirement of argininosuccinate lyase for systemic nitric oxide production. Nat. Med. 17 , 1619–1626 (2011).
Serghides, L. et al. Inhaled nitric oxide reduces endothelial activation and parasite accumulation in the brain, and enhances survival in experimental cerebral malaria. PLoS ONE 6 , e27714 (2011).
Mack, A.K. et al. Sodium nitrite promotes regional blood flow in patients with sickle cell disease: a phase I/II study. Br. J. Haematol. 142 , 971–978 (2008).
Wondji, C.S. et al. Impact of pyrethroid resistance on operational malaria control in Malawi. Proc. Natl. Acad. Sci. USA 109 , 19063–19070 (2012).
Chong, C.R., Chen, X., Shi, L., Liu, J.O. & Sullivan, D.J. Jr. A clinical drug library screen identifies astemizole as an antimalarial agent. Nat. Chem. Biol. 2 , 415–416 (2006).
Weisman, J.L. et al. Searching for new antimalarial therapeutics amongst known drugs. Chem. Biol. Drug Des. 67 , 409–416 (2006).
Guiguemde, W.A. et al. Chemical genetics of Plasmodium falciparum . Nature 465 , 311–315 (2010).
Gamo, F.J. et al. Thousands of chemical starting points for antimalarial lead identification. Nature 465 , 305–310 (2010).
Rottmann, M. et al. Spiroindolones, a potent compound class for the treatment of malaria. Science 329 , 1175–1180 (2010).
Yuan, J. et al. Genetic mapping of targets mediating differential chemical phenotypes in Plasmodium falciparum . Nat. Chem. Biol. 5 , 765–771 (2009).
van Pelt-Koops, J.C. et al. The spiroindolone drug candidate NITD609 potently inhibits gametocytogenesis and blocks Plasmodium falciparum transmission to anopheles mosquito vector. Antimicrob. Agents Chemother. 56 , 3544–3548 (2012).
Peatey, C.L., Spicer, T.P., Hodder, P.S., Trenholme, K.R. & Gardiner, D.L. A high-throughput assay for the identification of drugs against late-stage Plasmodium falciparum gametocytes. Mol. Biochem. Parasitol. 180 , 127–131 (2011).
Buchholz, K. et al. A high-throughput screen targeting malaria transmission stages opens new avenues for drug development. J. Infect. Dis. 203 , 1445–1453 (2011).
Eastman, R.T. et al. A class of tricyclic compounds blocking malaria oocyst development and transmission. Antimicrob. Agents Chemother. 57 , 425–435 (2013).
Burstein, E.S. et al. Integrative functional assays, chemical genomics and high throughput screening: harnessing signal transduction pathways to a common HTS readout. Curr. Pharm. Des. 12 , 1717–1729 (2006).
Goldstein, D.M., Gray, N.S. & Zarrinkar, P.P. High-throughput kinase profiling as a platform for drug discovery. Nat. Rev. Drug Discov. 7 , 391–397 (2008).
Coteron, J.M. et al. Structure-guided lead optimization of triazolopyrimidine-ring substituents identifies potent Plasmodium falciparum dihydroorotate dehydrogenase inhibitors with clinical candidate potential. J. Med. Chem. 54 , 5540–5561 (2011).
Biagini, G.A. et al. Generation of quinolone antimalarials targeting the Plasmodium falciparum mitochondrial respiratory chain for the treatment and prophylaxis of malaria. Proc. Natl. Acad. Sci. USA 109 , 8298–8303 (2012).
Yeh, E. & DeRisi, J.L. Chemical rescue of malaria parasites lacking an apicoplast defines organelle function in blood-stage Plasmodium falciparum . PLoS Biol. 9 , e1001138 (2011).
Ralph, S.A. et al. Tropical infectious diseases: metabolic maps and functions of the Plasmodium falciparum apicoplast. Nat. Rev. Microbiol. 2 , 203–216 (2004).
Nguitragool, W. et al. Malaria parasite clag3 genes determine channel-mediated nutrient uptake by infected red blood cells. Cell 145 , 665–677 (2011).
Nakazawa, S., Kanbara, H. & Aikawa, M. Plasmodium falciparum : recrudescence of parasites in culture. Exp. Parasitol. 81 , 556–563 (1995).
Nakazawa, S., Maoka, T., Uemura, H., Ito, Y. & Kanbara, H. Malaria parasites giving rise to recrudescence in vitro . Antimicrob. Agents Chemother. 46 , 958–965 (2002).
Thapar, M.M., Gil, J.P. & Bjorkman, A. In vitro recrudescence of Plasmodium falciparum parasites suppressed to dormant state by atovaquone alone and in combination with proguanil. Trans. R. Soc. Trop. Med. Hyg. 99 , 62–70 (2005).
Veiga, M.I. et al. Antimalarial exposure delays Plasmodium falciparum intra-erythrocytic cycle and drives drug transporter genes expression. PLoS ONE 5 , e12408 (2010).
Hoshen, M.B., Na-Bangchang, K., Stein, W.D. & Ginsburg, H. Mathematical modelling of the chemotherapy of Plasmodium falciparum malaria with artesunate: postulation of 'dormancy', a partial cytostatic effect of the drug, and its implication for treatment regimens. Parasitology 121 , 237–246 (2000).
Witkowski, B. et al. Increased tolerance to artemisinin in Plasmodium falciparum is mediated by a quiescence mechanism. Antimicrob. Agents Chemother. 54 , 1872–1877 (2010).
Teuscher, F. et al. Artemisinin-induced dormancy in Plasmodium falciparum : duration, recovery rates, and implications in treatment failure. J. Infect. Dis. 202 , 1362–1368 (2010).
Codd, A., Teuscher, F., Kyle, D.E., Cheng, Q. & Gatton, M.L. Artemisinin-induced parasite dormancy: a plausible mechanism for treatment failure. Malar. J. 10 , 56 (2011).
Tucker, M.S., Mutka, T., Sparks, K., Patel, J. & Kyle, D.E. Phenotypic and genotypic analysis of in vitro –selected artemisinin-resistant progeny of Plasmodium falciparum . Antimicrob. Agents Chemother. 56 , 302–314 (2012).
Nosten, F. Waking the sleeping beauty. J. Infect. Dis. 202 , 1300–1301 (2010).
Cheng, Q., Kyle, D.E. & Gatton, M.L. Artemisinin resistance in Plasmodium falciparum : a process linked to dormancy? Int. J. Parasitol. Drugs Drug Resist. 2 , 249–255 (2012).
LaCrue, A.N., Scheel, M., Kennedy, K., Kumar, N. & Kyle, D.E. Effects of artesunate on parasite recrudescence and dormancy in the rodent malaria model Plasmodium vinckei . PLoS ONE 6 , e26689 (2011).
Download references
Acknowledgements
This review was supported by the Division of Intramural Research, National Institute of Allergy and Infectious Diseases, US National Institutes of Health. We thank S.K. Pierce, S. Desai and C. Pola for critical comments and figure design.
Author information
Authors and affiliations.
Laboratory of Malaria and Vector Research, National Institute of Allergy and Infectious Diseases, Rockville, Maryland, USA
Louis H Miller, Hans C Ackerman, Xin-zhuan Su & Thomas E Wellems
You can also search for this author in PubMed Google Scholar
Corresponding author
Correspondence to Louis H Miller .
Ethics declarations
Competing interests.
The authors declare no competing financial interests.
Rights and permissions
Reprints and permissions
About this article
Cite this article.
Miller, L., Ackerman, H., Su, Xz. et al. Malaria biology and disease pathogenesis: insights for new treatments. Nat Med 19 , 156–167 (2013). https://doi.org/10.1038/nm.3073
Download citation
Received : 18 April 2012
Accepted : 17 December 2012
Published : 06 February 2013
Issue Date : February 2013
DOI : https://doi.org/10.1038/nm.3073
Share this article
Anyone you share the following link with will be able to read this content:
Sorry, a shareable link is not currently available for this article.
Provided by the Springer Nature SharedIt content-sharing initiative
This article is cited by
Distinct transcriptomic signatures define febrile malaria depending on initial infective states, asymptomatic or uninfected.
- Kelvin M. Kimenyi
- Mercy Y. Akinyi
- Lynette Isabella Ochola-Oyier
BMC Infectious Diseases (2024)
Piperine Enhances Antimalarial Activity of Methyl Gallate and Palmatine Combination
- Adegbenro P. Adegunloye
- Joseph O. Adebayo
Acta Parasitologica (2024)
Malaria epidemiological characteristics and control in Guangzhou, China, 1950–2022
- Yuehua Chen
- Yuehong Wei
Malaria Journal (2023)
Association of severe malaria with cognitive and behavioural outcomes in low- and middle-income countries: a meta-analysis and systematic review
- Andrew Sentoogo Ssemata
- Ann Jacquelline Nakitende
- Megan S. McHenry
The effect of dosage on the protective efficacy of whole-sporozoite formulations for immunization against malaria
- Diana Moita
- Catarina Rôla
- Miguel Prudêncio
npj Vaccines (2023)
Quick links
- Explore articles by subject
- Guide to authors
- Editorial policies
Sign up for the Nature Briefing newsletter — what matters in science, free to your inbox daily.


An official website of the United States government
The .gov means it’s official. Federal government websites often end in .gov or .mil. Before sharing sensitive information, make sure you’re on a federal government site.
The site is secure. The https:// ensures that you are connecting to the official website and that any information you provide is encrypted and transmitted securely.
- Publications
- Account settings
- My Bibliography
- Collections
- Citation manager
Save citation to file
Email citation, add to collections.
- Create a new collection
- Add to an existing collection
Add to My Bibliography
Your saved search, create a file for external citation management software, your rss feed.
- Search in PubMed
- Search in NLM Catalog
- Add to Search
Malaria parasite prevalence among migrants: a systematic review and meta-analysis
Collaborators.
- Malaria Migrant Collaborative group : Azucena Rodríguez-Cuadrado , Begoña Monge-Maillo , Cristina Bocanegra , Fernando Salvador , Ines Oliveira , Joaquin Salas-Coronas , Maria Dolores Corbacho-Loarte
Affiliations
- 1 Division of Infectious Diseases, Department of Medicine Solna, Karolinska Institutet, Stockholm, Sweden; Department of Infectious Diseases, Karolinska University Hospital, Stockholm, Sweden; Migrant Health Research group, Barcelona Institute for Global Health (ISGlobal, University of Barcelona), Barcelona, Spain; CIBERINFEC, (CIBER de Enfermedades Infecciosas), Instituto de Salud Carlos III, Centro de Investigación Biomédica en Red de Enfermedades Infecciosas, Madrid, Spain. Electronic address: [email protected].
- 2 Department of Infectious Tropical Diseases and Microbiology, Scientific Institute for Research, Hospitalization and Healthcare (IRCCS) Sacro Cuore-Don Calabria Hospital, Negrar di Valpolicella, Verona, Italy.
- 3 Division of Infectious Diseases, Department of Medicine Solna, Karolinska Institutet, Stockholm, Sweden.
- 4 Migrant Health Research group, Barcelona Institute for Global Health (ISGlobal, University of Barcelona), Barcelona, Spain.
- 5 Division of Infectious Diseases, Department of Medicine Solna, Karolinska Institutet, Stockholm, Sweden; Department of Infectious Diseases, Västerås Hospital, Västerås, Sweden.
- 6 Division of Infectious Diseases, Department of Medicine Solna, Karolinska Institutet, Stockholm, Sweden; Department of Infectious Diseases, Karolinska University Hospital, Stockholm, Sweden.
- PMID: 37739263
- DOI: 10.1016/j.cmi.2023.09.010
Background: Asymptomatic malaria infections are highly prevalent in endemic areas.
Objectives: This systematic review aimed to estimate the pooled prevalence of malaria parasites in migrants screened in non-endemic areas.
Data sources: MEDLINE-Ovid, EMBASE, Web of Science, Global Health, Lilacs, Cochrane, and MedRxiv.
Study eligibility criteria: Cross-sectional studies and observational prospective or retrospective cohort studies conducted in Europe, USA, Canada, Australia, or New Zealand regardless of language or publication status. Studies should include prevalence data on malaria in migrants that were recruited through a systematic screening approach. We excluded studies where people were tested because of malaria symptoms.
Participants: Migrant individuals exposed to malaria infection ASSESSMENT OF RISK OF BIAS: A standardized and validated appraisal instrument was used for studies reporting prevalence data (Joanna Briggs Institute Manual for Evidence Synthesis).
Methods of data synthesis: Pooled estimates of the parasite prevalence by PCR, microscopy, and rapid diagnostic test (RDT) were calculated with a random-effects model. Heterogeneity was explored by stratification by age, region of origin, period of study, and quality of studies.
Results: Of 1819 studies retrieved, 23 studies were included with in total 4203 participant PCR data, 3186 microscopy and 4698 RDT data, respectively. Migrants from sub-Saharan Africa had a malaria parasite prevalence of 8.3% (95% CI 5.1-12.2) by PCR, 4.3% (1.5-8.2) by RDT, and 3.1% (0.7-6.8) by microscopy. For migrants from Asia and Latin America, the prevalence with PCR was 0% (0.0-0.08) and 0.4% (0.0-1.8), respectively. Migrants from the Central African Region had the highest PCR prevalence (9.3% [6.0-13.0]), followed by West African migrants (2.0% [0.0-7.7]). Restricting the analysis to sub-Saharan Africa migrants arriving to the host country within the previous year, the PCR-based prevalence was 11.6% (6.9-17.4).
Conclusion: We provide estimates on the malaria parasite prevalence in migrants in non-endemic setting. Despite heterogeneity between settings, these findings can contribute to inform screening strategies and guidelines targeting malaria in migrants.
Keywords: Malaria; Meta-analysis; Migrant; Plasmodium; Prevalence; Systematic review.
Copyright © 2023 The Author(s). Published by Elsevier Ltd.. All rights reserved.
PubMed Disclaimer
Similar articles
- Malaria parasite prevalence in Sub-Saharan African migrants screened in Sweden: a cross-sectional study. Wångdahl A, Bogale RT, Eliasson I, Broumou I, Faroogh F, Lind F, Vashchuk G, Hildell A, Franson S, Hallberg E, Grip I, Nordling I, Gervin A, Kaitoly S, Tekleab B, Wyss K, Requena-Méndez A, Hertting O, Färnert A. Wångdahl A, et al. Lancet Reg Health Eur. 2023 Jan 13;27:100581. doi: 10.1016/j.lanepe.2022.100581. eCollection 2023 Apr. Lancet Reg Health Eur. 2023. PMID: 37069854 Free PMC article.
- Folic acid supplementation and malaria susceptibility and severity among people taking antifolate antimalarial drugs in endemic areas. Crider K, Williams J, Qi YP, Gutman J, Yeung L, Mai C, Finkelstain J, Mehta S, Pons-Duran C, Menéndez C, Moraleda C, Rogers L, Daniels K, Green P. Crider K, et al. Cochrane Database Syst Rev. 2022 Feb 1;2(2022):CD014217. doi: 10.1002/14651858.CD014217. Cochrane Database Syst Rev. 2022. PMID: 36321557 Free PMC article.
- The prevalence of anxiety, depression, and post-traumatic stress disorder among African migrants: A systematic review and meta-analysis. James PB, Renzaho AMN, Mwanri L, Miller I, Wardle J, Gatwiri K, Lauche R. James PB, et al. Psychiatry Res. 2022 Nov;317:114899. doi: 10.1016/j.psychres.2022.114899. Epub 2022 Oct 10. Psychiatry Res. 2022. PMID: 36252417 Review.
- Prevalence of strongyloidiasis and schistosomiasis among migrants: a systematic review and meta-analysis. Asundi A, Beliavsky A, Liu XJ, Akaberi A, Schwarzer G, Bisoffi Z, Requena-Méndez A, Shrier I, Greenaway C. Asundi A, et al. Lancet Glob Health. 2019 Feb;7(2):e236-e248. doi: 10.1016/S2214-109X(18)30490-X. Lancet Glob Health. 2019. PMID: 30683241
- Prevalence of Chagas disease in Latin-American migrants living in Europe: a systematic review and meta-analysis. Requena-Méndez A, Aldasoro E, de Lazzari E, Sicuri E, Brown M, Moore DA, Gascon J, Muñoz J. Requena-Méndez A, et al. PLoS Negl Trop Dis. 2015 Feb 13;9(2):e0003540. doi: 10.1371/journal.pntd.0003540. eCollection 2015 Feb. PLoS Negl Trop Dis. 2015. PMID: 25680190 Free PMC article. Review.
- Therapeutic Potential of Marine-Derived Cyclic Peptides as Antiparasitic Agents. Ribeiro R, Costa L, Pinto E, Sousa E, Fernandes C. Ribeiro R, et al. Mar Drugs. 2023 Nov 25;21(12):609. doi: 10.3390/md21120609. Mar Drugs. 2023. PMID: 38132930 Free PMC article. Review.
Publication types
- Search in MeSH
Related information
Linkout - more resources, full text sources.
- Elsevier Science
- Genetic Alliance
- MedlinePlus Health Information
Research Materials
- NCI CPTC Antibody Characterization Program
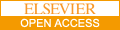
- Citation Manager
NCBI Literature Resources
MeSH PMC Bookshelf Disclaimer
The PubMed wordmark and PubMed logo are registered trademarks of the U.S. Department of Health and Human Services (HHS). Unauthorized use of these marks is strictly prohibited.
Loading metrics
Open Access
Pearls provide concise, practical and educational insights into topics that span the pathogens field.
See all article types »
A new landscape for malaria vaccine development
Affiliations Center for Vaccine Development and Global Health, University of Maryland School of Medicine, Baltimore, Maryland, United States of America, Molecular Microbiology and Immunology Program, Graduate Program in Life Sciences, University of Maryland School of Medicine, Baltimore, Maryland, United States of America

* E-mail: [email protected]
Affiliation Center for Vaccine Development and Global Health, University of Maryland School of Medicine, Baltimore, Maryland, United States of America
- Alexander J. Laurenson,
- Matthew B. Laurens
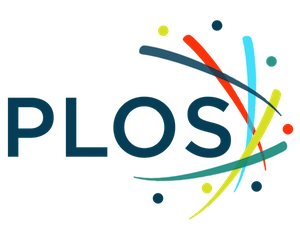
Published: June 27, 2024
- https://doi.org/10.1371/journal.ppat.1012309
- Reader Comments
Citation: Laurenson AJ, Laurens MB (2024) A new landscape for malaria vaccine development. PLoS Pathog 20(6): e1012309. https://doi.org/10.1371/journal.ppat.1012309
Editor: Audrey Ragan Odom John, Children’s Hospital of Philadelphia, UNITED STATES
Copyright: © 2024 Laurenson, Laurens. This is an open access article distributed under the terms of the Creative Commons Attribution License , which permits unrestricted use, distribution, and reproduction in any medium, provided the original author and source are credited.
Funding: AJL is supported by institutional funds via University of Maryland, Baltimore, Graduate Program in Life Sciences, Graduate Research Assistantship. MBL is supported by grants and contracts to his institution from the U.S. National Institutes of Health (UM1AI148689 and U01AI155300), Bill & Melinda Gates Foundation (INV-030857), Bill & Melinda Gates Medical Research Institute, and BioNTech, SE. The funders had no role in study design, data collection and analysis, decision to publish, or preparation of the manuscript.
Competing interests: AL and ML are listed on a pending International Patent Application PCT/US2023/077892.
On October 6, 2021, the World Health Organization (WHO) recommended the first vaccine against malaria to prevent Plasmodium falciparum malaria in children living in areas with moderate to high transmission [ 1 ], a watershed moment in child health. This historic event was informed by results of WHO pilot implementation of the RTS,S vaccination in Ghana, Kenya, and Malawi, that documented feasibility to deliver through routine immunization systems, capacity to increase equity to malaria prevention, a strong safety profile, significant reduction in severe malaria, and high cost effectiveness [ 2 ]. More recent analysis of the RTS,S pilot implementation results demonstrated 13% all-cause mortality reduction even in the presence of only moderate vaccine coverage [ 3 ]. Enthusiasm for RTS,S implementation in endemic countries has resulted in 18 country approvals to date for Gavi support for vaccine introduction, and current limited supply through 2025 was allocated to 12 of these countries [ 4 ].
Two years later, the WHO recommended a second malaria vaccine R21/Matrix-M (R21) on October 2, 2023 [ 5 ]. Like RTS,S, R21 generates immunity to P . falciparum circumsporozoite protein (CSP). A recent Phase 3 clinical trial of R21 in children 5 to 36 months of age demonstrated 75% efficacy at 2 sites with seasonal transmission and 68% efficacy at 3 sites with perennial transmission [ 6 ]. While RTS,S and R21 have not been compared head-to-head, they are expected to perform similarly and substantially impact malaria morbidity and mortality in endemic areas. R21 has a significant cost advantage at US $2 to 4 per dose and is expected to fill the huge demand-supply gap.
Now, with 2 high-impact malaria vaccines becoming available, how has this milestone influenced malaria vaccine research and development efforts? This article aims to explain more about the current landscape of malaria vaccine development.
Question 1. Why are more candidate vaccines needed for malaria?
Although 2 vaccines are recommended, neither meet the desired efficacy and durability for an optimal malaria vaccine. WHO’s preferred product characteristics for a malaria vaccine target a 90% reduction in blood stage infection and clinical malaria over 12 months [ 7 ]. When administered seasonally alongside seasonal malaria chemoprophylaxis as a three-dose series, during 12 months of follow-up, RTS,S demonstrated 72% efficacy [ 8 ], and R21 demonstrated 75% efficacy [ 6 ]. Vaccine-induced immunity wanes over time, which is somewhat mitigated by a fourth and possibly fifth annual booster. Next-generation vaccines that provide even higher efficacy can achieve greater public health impact, possibly requiring fewer doses and no annual booster. Such vaccines could increase individual protection, decrease vaccine delivery system demands, improve cost effectiveness, and further increase equity to malaria prevention.
Similar to COVID-19 vaccine development, multiple vaccine products are needed to ensure vaccine supply. Though not always foreseeable or desirable, any manufacturing or safety concern could surface and indefinitely remove a vaccine from use and necessitate use of an alternate product. Plans to produce malaria vaccines in India and sub-Saharan Africa will increase capacity to meet the current demand. Having multiple products manufactured in different facilities would help to ensure replacement product is available and to provide endemic countries with uninterrupted vaccine access.
Question 2. What might next-generation malaria vaccines look like?
Many next-generation malaria vaccines are currently in clinical testing ( Table 1 ). Some use novel approaches including live attenuated sporozoite inoculations, RNA-based platforms, and a combination of existing P . falciparum CSP-based vaccines with antigens from other stages of the parasite life cycle. Live attenuated sporozoite approaches build on human studies that demonstrated 90% protection against malaria infection among adults immunized with radiation-attenuated sporozoites administered via at least 1,000 infected bites [ 9 ]. Subsequent advances in cryopreservation of live sporozoites has led to whole organism vaccination regimens tested in the US, Europe, and sub-Saharan Africa, which all demonstrate protection against P . falciparum malaria [ 10 ]. Researchers are now planning trials of late liver stage-arresting, replication competent (LARC), genetically attenuated P . falciparum sporozoite vaccines that build on safety, immunogenicity, and efficacy demonstrated using previous generation whole sporozoite vaccines but multiply asexually in the liver and thus provide a prolonged stimulation of infection-blocking immune responses.
- PPT PowerPoint slide
- PNG larger image
- TIFF original image
https://doi.org/10.1371/journal.ppat.1012309.t001
Based on the recent success of COVID-19 vaccine development, mRNA-lipid nanoparticle technology is being employed for malaria vaccines in 2 human studies ( Table 1 ). mRNA-based vaccines provide advantage as they can be manufactured quickly, are safe and effective for young infants and pregnant women, and can code for multiple antigens to strengthen the immune response. Disadvantages include side effects, though these are generally mild and temporary. The first mRNA-based malaria clinical trial tests a single RNA construct encoding part of the P . falciparum circumsporozoite protein (CSP), and the second tests a combination of 3 distinct RNAs—the full P . falciparum CSP and 2 conserved segments of liver stage-expressed proteins—with plans for controlled human malaria infection to determine preliminary vaccine efficacy. Other promising RNA-based malaria vaccine strategies are in preclinical development [ 11 – 14 ].
Another strategy for malaria vaccines focuses on improving RTS,S and R21 efficacy in preventing disease by adding a separate vaccine antigen targeting the parasite’s erythrocytic cycle so a single product would provide both pre-erythrocytic liver stage protection and erythrocytic efficacy against parasitic escape. One such strategy combining R21 with the blood stage antigen reticulocyte-binding protein homolog 5 (RH5) is already underway [ 15 ].
Question 3. How will computational biology inform next-generation malaria vaccines?
Most current malaria vaccine target antigens were discovered by identifying immune responses in following malaria infection, yet few have demonstrated efficacy in clinical studies. Reasons for vaccine failure include antigenic variation, off-target antibody responses diluting intended protective responses, and short durability of immunity [ 4 ]. Novel bioinformatics tools can overcome these obstacles by leveraging parasite and human genomic data to strategically identify candidate vaccine targets that generate precise and accurate immunity, and to overcome parasite diversity.
To optimize immunogenicity and targeted immunity, computational techniques such as 3D protein modeling can predict conformation-dependent immune responses to malaria proteins, which allows researchers to identify parasite gene loci that are susceptible to immune escape from vaccine-induced protection [ 16 ]. In addition, integrating known local HLA polymorphism and parasite population sequence data from endemic regions to identify T cell epitopes recognizable by common HLA alleles optimizes vaccine design, ensuring results are directly applicable to target populations.
Despite P . falciparum ’s enormous antigenic diversity, comprehensive analyses of parasite genomic and transcriptomic data collected in endemic areas can identify genomic regions under positive selection pressure to remain conserved [ 17 ]. These antigens serve as ideal candidate vaccines. Moreover, parasite transcriptomic profile analysis pinpoints essential proteins consistently expressed during distinct life cycle stages that can also serve as vaccine targets [ 17 ]. Advanced characterization of P . falciparum ’s complex genome using a combined set of approaches can provide a more credible and well-informed selection of target regions as candidate vaccine antigens for development.
A pipeline approach that incorporates high-throughput analyses in sequence can predict conserved and positively selected antigenic regions that elicit successful and protective immune responses, circumventing traditional preclinical experimentation that is costly and time-consuming. With experimentally validated bioinformatic predictive tools informed by genomic datasets, resources are deployed precisely and efficiently, thus accelerating antigen discovery for preclinical testing.
Question 4. How will next-generation malaria vaccines be down-selected?
RTS,S underwent a lengthy 35-year development from creation in 1987 [ 18 ] to 2021 when the WHO recommended it for use [ 1 ]. CSP was identified as a target of the immune response generated by radiation-attenuated sporozoites, and epitope mapping led to development of a subunit vaccine that demonstrated protection against Controlled Human Malaria Infection (CHMI). RTS,S was then tested with multiple adjuvants, in rhesus and then in human clinical trials with CHMI in malaria-naïve adults and subsequently in malaria-exposed adults and then children and infants living in endemic areas [ 18 ]. As no known correlate of RTS,S-induced protection against P . falciparum was identified, efficacy studies in the target population of children living in endemic areas were required to assess RTS,S impact.
Now, with data from multiple clinical trials of RTS,S, recent advances in our understanding of vaccine-induced immunity to P . falciparum malaria, and refinement of preclinical models, it is possible to use mouse models to improve existing CSP-based vaccines [ 19 ]. Adjuvants can now be carefully selected based on the desired effector function, [ 20 ] obviating the need for large CHMI and/or efficacy studies to optimize adjuvant selection. Cryo-electron microscopy has advanced understanding of CSP-based structures underlying high antibody avidity and potency needed for an effective vaccine [ 21 ]. As regulatory bodies and experienced clinical trial centers exist in malaria endemic areas, candidate next-generation vaccines ready for human testing can be trialed in first-in-human studies with CHMI in endemic countries, lessening the need for initial testing in the US and Europe and potentially shortening time needed for clinical development. Overall, these advances will facilitate efficient testing of improved CSP-based vaccines.
Question 5. What about vaccines that block transmission?
Vaccines that prevent malaria transmission are needed to achieve elimination goals. A highly effective pre-erythrocytic vaccine would completely prevent parasite erythrocytic development and thus halt onward transmission, though developing a vaccine with 100% efficacy may not be feasible. RTS,S and R21 are pre-erythrocytic vaccines that incompletely prevent blood stage infection, thus improving malaria morbidity and mortality. These vaccines address the first 2 WHO strategic priorities for malaria vaccines to prevent human blood-stage infection at the individual level and to reduce morbidity and mortality in individuals at risk in malaria-endemic areas [ 7 ]. However, they do not address the third WHO strategic priority to reduce parasite transmission and incidence of human infection in the community [ 22 ]. Malaria vaccines that reduce transmission exclusively would not provide health benefit to an individual but would significantly impact malaria elimination efforts at the community and regional levels.
Vaccines targeting P . falciparum antigens expressed during parasite sexual development in the mosquito midgut represent a promising approach to prevent malaria transmission to mosquitoes, blocking onward transmission to humans. As these antigens are not seen by the human immune system during parasite development, they are not targets of naturally acquired immunity. Transmission-blocking vaccines can induce antibodies that are subsequently ingested by the mosquito vector during a blood meal and that act directly on parasites. Such vaccines are based on parasite antigens expressed in the mosquito midgut, including Pfs230 and Pfs25 [ 23 ], and Pfs48/45 [ 24 ]. Transmission-blocking vaccines could be administered as a standalone product or combined with a pre-erythrocytic or erythrocytic vaccine to provide both individual and community benefit.
As clinical trials of transmission-blocking vaccines that measure community transmission as an outcome would require a large number of participants exposed to an investigational product to measure efficacy, immunogenicity studies can be used as proxies. In addition to measurements of antibody against the vaccine antigen, serum functional activity against parasite sexual stage development is measured using a standard membrane feeding assay, where mosquitoes feed on cultured gametocytes in the presence of serum and are then observed for parasite oocyst development within each mosquito [ 25 ]. Direct skin feeding assays can also be used where female Anopheles are placed in a mesh container and allowed to feed directly at the skin surface of a vaccinated participant, then later dissected to assess for parasite oocyst development [ 23 ]. Results of these functional assays inform clinical development, though no transmission-blocking vaccine has progressed beyond Phase 2 testing to date.
Conclusions
The first 2 malaria vaccines recommended by the WHO in 2021 and 2023 may have arrived just in time, as current malaria case counts remain essentially unchanged since 2015, reports of first-line antimalarial resistance are becoming more common, and climate change threatens recent advances in malaria control. The advent of these vaccines has been met with strong public interest in vaccination as a means to tackle malaria, and signals that future improvements in malaria vaccines will likely achieve similar high demand and uptake. Next-generation vaccines are needed to provide enhanced and sustained efficacy that will improve child health, increase educational outcomes for children, save lives, and advance elimination efforts. Preclinical work to define new and improved vaccine antigens can be informed by computational biology pipelines to increase efficiency. While multiple interventions are needed to control malaria in endemic areas, high-impact interventions that prevent the most illnesses and deaths with available resources are a priority. Malaria vaccines represent a high-impact intervention that can reduce clinical disease, prevent severe malaria illness, decrease hospitalizations, and improve child survival [ 3 ]. Vaccines epitomize a viable strategy that can be furthered and advanced through continued research and innovation to accelerate malaria elimination efforts and shrink existing health disparities in resource-limited areas, paving the way toward a malaria-free future.
- 1. WHO recommends groundbreaking malaria vaccine for children at risk. World Health Organization; 2021 Oct.
- View Article
- Google Scholar
- PubMed/NCBI
- 4. Global Malaria Programme (GMP), World Health Organization. World Malaria Report 2023. 2023.
- 5. WHO recommends R21/Matrix-M vaccine for malaria prevention in updated advice on immunization. World Health Organization; 2023 Oct.
- 7. Malaria vaccines: preferred product characteristics and clinical development considerations. World Health Organization; 2022 Sep.
- 15. University of Oxford. A Study to Assess the Experimental Malaria Vaccines RH5.2-VLP and R21 [Internet]. 2023 Jul. Report No.: NCT05357560. Available from: https://clinicaltrials.gov/study/NCT05357560 .
- Open access
- Published: 17 January 2023
Impact of insecticide resistance on malaria vector competence: a literature review
- Pierre Fongho Suh 1 , 2 ,
- Emmanuel Elanga-Ndille 3 ,
- Magellan Tchouakui 3 ,
- Maurice Marcel Sandeu 3 , 4 ,
- Darus Tagne 1 , 5 ,
- Charles Wondji 1 , 6 &
- Cyrille Ndo 1 , 7
Malaria Journal volume 22 , Article number: 19 ( 2023 ) Cite this article
7724 Accesses
17 Citations
11 Altmetric
Metrics details
Since its first report in Anopheles mosquitoes in 1950s, insecticide resistance has spread very fast to most sub-Saharan African malaria-endemic countries, where it is predicted to seriously jeopardize the success of vector control efforts, leading to rebound of disease cases. Supported mainly by four mechanisms (metabolic resistance, target site resistance, cuticular resistance, and behavioural resistance), this phenomenon is associated with intrinsic changes in the resistant insect vectors that could influence development of invading Plasmodium parasites. A literature review was undertaken using Pubmed database to collect articles evaluating directly or indiretly the impact of insecticide resistance and the associated mechanisms on key determinants of malaria vector competence including sialome composition, anti- Plasmodium immunity, intestinal commensal microbiota, and mosquito longevity. Globally, the evidence gathered is contradictory even though the insecticide resistant vectors seem to be more permissive to Plasmodium infections. The actual body of knowledge on key factors to vectorial competence, such as the immunity and microbiota communities of the insecticide resistant vector is still very insufficient to definitively infer on the epidemiological importance of these vectors against the susceptible counterparts. More studies are needed to fill important knowledge gaps that could help predicting malaria epidemiology in a context where the selection and spread of insecticide resistant vectors is ongoing.
Malaria is the biggest killer among vector-borne diseases [ 1 ] and has claimed the lives of milllions of people over centuries [ 2 ]. In 2020, 241 million cases were reported leading to 627,000 deaths. The African region has paid the highest tributes with 96% of all deaths [ 3 ]. Malaria disease is caused by Plasmodium parasites, which are transmitted to humans by the bites of infected female mosquitoes of the genus Anopheles [ 4 ]. In Africa, Plasmodium falciparum is the most epidemiologically important of malaria parasites infecting humans [ 5 ], and Anopheles gambiae , Anopheles coluzzii , Anopheles funestus and Anopheles arabiensis are the dominant vector species [ 6 ].
Malaria control includes medical treatment of cases and protective measures against the vectors to prevent and/or limit contacts with human hosts during which transmission occurs. The control of mosquito populations on a large scale using insecticide-treated nets (ITNs) and indoor residual spraying, associated with increase case management, has led to a remarkable reduction in malaria burden from 81.1 cases per 1000 population in 2000 to 58.9 in 2015 [ 3 ]. After this period, the impact of control efforts on malaria burden have dwindled, coinciding with the spread of insecticide resistant vectors across most endemic countries [ 3 , 7 ]. Resistance of Anopheles mosquitoes to insecticides, reported for the first time in Africa in the 1950s [ 7 ], concerns four main classes of insecticides used in public health for vector control purposes, namely pyrethroids, organochlorines, organophosphates and carbamates [ 7 , 8 ]. There are four mechanisms deployed by mosquitoes to become insensitive to the insecticides, including by order of importance (1) degradation of insecticide molecules by detoxification enzymes (metabolic resistance), (2) modification of the target affinity of the insecticide (target site resistance), (3) reduced penetration of the insecticide (cuticular resistance) and, (4) avoidance of insecticide-treated surfaces (behavioural resistance). Of these four mechanisms target site and metabolic resistances are most likely to lead to control failure [ 9 ].
In target site resistance, a change (leucine changed to a phenylalanine or a serine at position 1014) occurring in the amino acid sequence of the voltage gate sodium channel (vgsc) leads to a reduced sensitivity of mosquitoes to pyrethroids and organochlorines. This phenotype is known as knock down resistance or kdr [ 10 , 11 ]. When the amino acid change (glycine replaced by serine at position 119) occurs in the neurotransmitter acetyl-cholinesterase, it occasions resistance to organophosphates and carbamates, termed ace-1 resistance [ 12 , 13 ]. About metabolic resistance, insecticide resistant mosquitoes increase the expression of detoxification enzymes, such as the cytochrome P450 monooxygenases, glutathione S-transferases (GSTs) and esterases, that eliminates xenobiotic compounds (including insecticides) before they reach their target. In another instance, an amino acid substitutions in the sequence of detoxification enzymes could modifiy its affinity with the insecticides in insect vectors [ 14 ]. For example, several cytochrome P450 genes ( CYP6P9a , CYP6P9b and CYP6M7 ) are involved in resistance to pyrethroids in the species An. funestus [ 15 , 16 ]; while a substitution of leucine by phenylalanine at position 119 in the epsilon class of GST ( GST2 - L119F) confers a cross-resistance to dichloro-diphenyl-trichloroethane (DDT) and pyrethroids in the same vector species [ 17 ].
Despite the widespread distribution of insecticide resistance, its impact on overall malaria epidemiology remains unclear and is currently a subject of intense debate. The evaluation of the potential impact of insecticide resistance on vectorial competence is therefore becoming an important and urgent research theme whose findings will help understanding whether it alters or enhances the permissiveness of malaria vectors to Plasmodium parasites, from its early stage (ookinete) to the infective form (sporozoite). In this review, the evidence of insecticide resistance impact on the infectivity of mosquitoes to Plasmodium was explored in the literature, and changes in intrinsic factors that could predict or explain the outcome of an infectious blood meal intake were broached. Finally, the knowledge gaps were pointed out.
Search strategy
A literature search was undertaken in the PubMed database to extract articles addressing the following themes: (1) Plasmodium infection in insecticide resistant malaria vectors, (2) sialome of insecticide resistant malaria vectors, (3) effect of insecticide resistance on the immunity of malaria vectors, (4) microbiota of insecticide resistant malaria vectors and infection, and (5) fitness cost of insecticide resistance in malaria vectors. The first search terms were “ Anopheles ” and “insecticide resistance” and they were associated with either “ Plasmodium infection”, “vector competence”, “salivary gland”, “sialome”, “microbiota”, “gene expression” or “longevity”. Additional articles were extracted from the references lists of the full publications. The search was done between February and August 2022 and there was no restriction regarding the date of publication of the articles. A total of 560 articles were obtained from the search. Articles that addressed insecticide resistance in Anopheles in a broad manner, and not in relation with either Plamodium infection, vector competence, sialome, or longevity were discarded. Therefore, 28 articles related to the themes mentioned above were selected and used for the review.
Malaria vector competence
Vector competence is the intrinsic ability of anopheline species or populations to allow the development of Plasmodium parasites from ookinete to infective sporozoites. When a mosquito takes an infectious blood meal from human, the gametocytes ingested begin their development in the midgut. The male gametocyte transform into eight microgametes after three rounds of mitosis, meanwhile the female gametocytes matures into macrogametes [ 4 ]. These cells fuse to form zygotes that thereafter change into ookinetes in the lumen of the intestine. The ookinetes then strive through the epithelium of the midgut and once in its basal side, transform into oocysts. The oocysts undergo several rounds of asexual multiplication (sporogony) leading to the production of thousands of haploid sporozoites in each oocyst. Mature occysts rupture and release sporozoites in the hemocoel, which immediately migrate to the salivary glands. The extrinsic incubation period of the parasite is about 14 days with the transition from ookinetes to mature oocysts having the highest duration (about 10 days) [ 18 , 19 ].
In mosquito host, Plasmodium face several immune-related bottlenecks deployed to prevent the successful transition from its early stage in the midgut to the sporozoite stage in the salivary glands [ 18 ]. The outcome of the parasite infection is reported to depend mainly on the mosquito- Plasmodium genetics adaptation [ 19 , 20 ]. Another very important factor that influences the above outcome is the compatibility of the duration of parasite development with the longevity of the mosquitoes [ 21 , 22 ]. Only species in which Plasmodium reaches infective form are referred to as competent vectors and could ensure malaria transmission. The impact of vector competence on the transmission of malaria can be estimated using Ro (Fig. 1 ), the basic reproductive number developed by McDonald in 1957. The McDonald model gives the threshold for a disease to persist or spread (Ro greater than 1) or to disappear (Ro less than 1) [ 23 ]. The Ro represents the number of individuals in a susceptible human population that are expected to get infected via a mosquito bite when a single infected individual is present in the population [ 24 , 25 ]. In the Ro equation, two parameters are related to vector competence: probability of mosquito infection (b) and mosquito longevity (p) (Fig. 1 ). Modifications of the values of components of this equation for a given vector population will cause either an augmentation or reduction in the transmission dynamics of the disease, leading probably to a change in the epidemiological profile of the locality concerned. It was established that an increase in b will increase the Ro, whereas a decrease in p will cause the opposite [ 26 ].
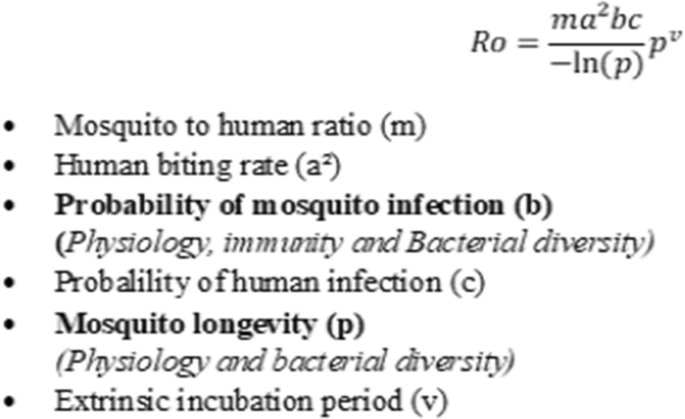
Basic reporductive number (Ro), Ross-MacDonald model. In bold, parameters of the vectorial competence influenced by insecticide resistance
Insecticide resistance and malaria vector infectivity to Plasmodium parasite
The rapid spread of insecticide resistance among malaria vectors accross endemic countries in the past decade have raised several questions among which that of knowing what is its impact on mosquito permissiveness to Plasmodium ? Only a limited number of studies have tried to elucidate this question [ 27 , 28 , 29 , 30 , 31 , 32 , 33 , 34 , 35 ]. These studies compared P. falciparum infection rates in resistant Anopheline vectors with susceptible ones, either caught in the field or experimentally infected (Table 1 ).
Anopheles gambiae strain bearing kdr resistance allele ( Vgsc - L 1014S) was found naturally more infected by sporozoites than the susceptible counterpart [ 27 ]. Similar findings were experimentally observed in the same species, as well as in Anopheles coluzzii [ 30 , 32 ]. Contrary to kdr resistance, An. gambiae with ace-1 resistance allele did not differ from individuals that have the wild type allele (not conferring insecticide resistance) on infection rate despite significantly higher oocyst prevalences were observed in the resistant strain [ 32 ]. More studies using field populations are needed to ascertain whether a lower longevity suspected by the author and/or other factors are involved.
Regarding metabolic resistance, recent breakthroughs in designing simple PCR-based assays to detect glutathione S-transferase (GST)-based and cytochrome P450-mediated resistance in An. funestus sensu stricto provided a unique opportunity to assess its impact on the mosquito’s ability to develop the parasites. The L119F- GSTe2 resistant genotypes of this species showed, in an experimental infection study, higher permissiveness to oocyst infections than susceptible ones [ 31 ]. Similarly, in naturally infected populations of the same species, homozygote L119F- GSTe2 genotypes were found more infected by sporozoites though no significant difference was found at the level of oocyst prevalence [ 28 ]. In other hands, Lo and Coetzee [ 36 ], infecting experimentally two selected sub-colonies of FUMOZ displaying different degree of pyrethroid resistance by Plasmodium berghei , found that the insecticide resistant colonies were less permissive to infection than the susceptible ones. No investigation has so far explored the relationship between P450s genes implicated in insecticide resistance and P. falciparum infection in An. funestus . Moreover, because of the absence of markers of metabolic resistance in An. gambiae sensu lato such studies are still lacking in these species.
Impact of insecticide resistance on mosquito sialome
Bloodsucking arthropods, like mosquitoes, have evolved saliva containing a mixture of pharmacologically active molecules that help them counteract the hemostatis and inflammatory responses of the vertebrate host during bites, thus facilitating blood meal intake [ 37 ]. However, the activity of these molecules goes beyond the scope of ensuring blood meal success, as they possibly influence the completion of Plasmodium development in the salivary gland of malaria vectors. Proteins secreted by the salivary gland belong to several families (D7, mucin, gSG1, gSG2, gSG6 peptide, gSG7, cE5, 8.2-kDa, 6.2-kDa, etc.) [ 38 ] whose function include (1) cytoskeletal and structural activities (2) digestion, (3) circadian rythm and chemosensory, (3) immunity, (4) metabolism and other [ 39 ]. The development of insecticide resistance in malaria vectors is accompanied by physiological changes [ 26 ] that may affect the sialome composition with consequences on the vector competence. Few studies have investigated changes in the sialome in the insecticide resistant vectors [ 40 , 41 ].
The secretory protein 100 kDa, which is encoded by Saglin (a cytoskeletal and structural gene present in An. gambiae salivary gland) was considered as the binding target of P. falciparum and P. berghei on salivary gland prior to penetration into the latter [ 42 ]. This protein was found down-regulated in ace-1 bearing An. gambiae strain, suggesting an impact on the vector infectivity to Plasmodium [ 43 ]. However, a recent study showed that the 100 kDa Protein is unevenly distributed on the salivary glands lobes. Its absence on the primary site of sporozoites occupancy in the salivary glands, the distal lateral lobes, implies that this protein may instead have a secondary role in the infection of the organ [ 44 , 45 , 46 ].
The D7 salivary family has been identified in malaria vectors among the most expressed proteins involved in the antihemostatic activity and probably in digestion of blood meal [ 47 , 48 , 49 , 50 ]. Elanga et al. [ 40 ] showed that two short forms of the D7 family genes ( D7r3 and D7r4 ) are over-expressed in pyrethroid resistant An. funestus ( L119F - GSTe2 ), whereas almost all D7 genes are under-expressed in pyrethroid resistant An. gambiae ( kdr , L1014F ). A comparable observation was made in insecticide resistant Culex quinquefasciatus ( ace-1 resistance) [ 51 ] as well as in two strains of Aedes aegypti (homozygotes resistant C1534 and G1016 kdr ) [ 52 ]. These findings show that insecticide resistance mechanism may affect the sialome composition differently.
Several immune proteins such as the anti-microbial peptides cecropin and defensin were found in the saliva of mosquitoes [ 39 , 53 ]. These immune proteins underscore the role of the salivary gland in the refractoriness of the Anopheles to infections [ 39 , 53 ]. The small number of studies that evaluated the impact of insecticide resistance alleles on salivary gland gene expression in mosquito vectors have not reported significant changes related to immune genes as compared with the susceptible counterparts [ 41 , 43 , 51 , 52 ], alluding that the resistant status to insecticide does not influence noticeably the immune component of the sialome. If these factors are indeed unchanged regardless of the mosquito allelic composition, nothing is known whether under infection the expression profile of these immune proteins will vary or not according to the mosquito genotype. Das et al. [ 39 ] and Djegbe et al. [ 51 ] demonstrated that salivary gland genes expression is influenced by blood meal intake and varies towards the period coinciding with the maturation of Plasmodium parasites in mosquitoes [ 54 ]. This evidence was not previously studied and should be taken into account in subsequent research works that aims at identifying differentially expressed genes of the salivary gland and elucidating their impact on the malaria vector competence.
Impact of insecticide resistance on vector immunity
When the infectious blood meal reaches the midgut of the female Anopheles , the immune system is deployed to prevent infections [ 20 ]. In the midgut, P. falciparum faces the peritrophic membrane, a physical barrier developed to prevent infections. It also protects against the damaging effects of the human blood factors like antibodies and regulates several digestive enzymes [ 55 , 56 ]. Enzymes such as trypsin 1 and 2, chymotrypsin, carboxypeptidase, aminopeptidase and serine protease are upregulated during digestion to cleave the large content of proteins in the blood meal [ 57 , 58 , 59 , 60 ]. These proteases are apparently involved in the elimination of Plasmodium infections [ 61 ]. Three studies attempted to elucidate the effect of insecticide resistance on vectors’ immunity [ 62 , 63 , 64 ]. Mitri et al. [ 62 ], in a study evaluating genes implicated in the infectivity of An. coluzzii , demonstrated that the kdr -bearing para gene which carries mutations of the voltage-gate sodium channel (confering insecticide resistance) is not associated with infection but rather the ClipC9 gene directing the synthesis of Serine protease. This suggest that the effect of the resistant character on refractoriness to infection may be due to genes other than that involved in resistance to insecticides, and which happen to be linked to it. The Serine protease plays an important role in the activation of the three major immune signaling pathways in mosquitoes: Toll, Imd and JAK/STAT [ 20 ], which cause the release of antimicrobial peptides (AMPs) notably defensins, cecropins, attacin, gambicin and AgSTAT-A, effective against malaria parasites infections. Vontas et al. [ 63 ], using pyrethroid and organochlorine resistant An. gambiae strains, showed that defensin and cecropin are upregulated after pre-exposure to permethrin. This study sugggests that insecticide resistant mosquitoes may be better equipped than susceptible ones to combat infections, but these two immune effectors alone may not be decisive in rendering the vector completely refractory to malaria infections as many other pathways activated concomitantly during parasitic invasion are altogether implicated in the outcome of a contamination [ 20 ].
In Culex pipiens which is vector of many pathogens including arboviruses [ 65 ], filarial worms [ 66 ], and protozoa [ 67 ], immune response was stimulated in an insecticide resistant field strain by injection of Lipopolysacharide (LPS) immune elicitor. As result, no difference was found in the expression of defensin and cecropin as compared to the control group; but only an increase in gambicin was recorded [ 68 ]. One point can be drawn from these results to infer what might happen in malaria vectors: Plasmodium infections may trigger the overexpresion of some immune factors while the other may have their expression either down regulated or unchanged.
The reactive oxygen species (ROS) produced by cellular metabolism are another class of effectors of the innate immunity that can negatively affect malaria parasites [ 69 , 70 ]. They kill the parasites through both lytic and melanization pathways [ 20 ]. Ingaham et al. [ 64 ] showed that An. coluzzii VK7 colony displaying kdr resistance mechanism, CYP6M6 and CYP6P3 metabolisers, had oxidoreductase overexpressed after sub-lethal exposure to deltamethrin, suggesting that this species could be more refractory to Plasmodium infection. At this point, it is necessary to verify whether under natural conditions, insecticide-resistant Anopheles mosquitoes will display an overexpression of ROS or not.
Cellular immune responses are carried out by varous type of hemocytes that eliminate pathogens by phagocytosis, lysis and melanization [ 20 ]. Organochlorines and organophosphate were found to affect differently the hemocytes abundance including granulocytes in the insect Rhynocoris kumarii [ 71 ]. In mosquitoes, studies are needed to ascertain the impact of insecticide resistance on cellular immunity and the resulting effect on the infectivity of resistant vector to malaria parasite. Regarding melanization of pathogens, it is lead by the phenoloxidase (PO) produced by Oenocytoids [ 72 , 73 ] and is regulated by serine protease inhibitors. In field-caught C. pipiens resistant to insecticide through an increase in detoxification (esterase) and target site mutation (ace-1), PO expression was equal to that of susceptible group [ 74 ], suggesting that some genes associated with immunity might not be affected by insecticide resistance character in mosquitoes. No studies have verified the effect of insecticide resistance on PO in malaria vectors.
Impact of insecticide resistance on commensal intestinal microbiota of malaria vectors
Bacteria, fungi and viruses colonize the gut, salivary glands and reproductive organs of the mosquitoes. These microorganisms are mainly acquired from the environnement and its composition is largely influenced by its aquatic breeding sites [ 75 , 76 ]. In addition, the microbiota composition is highly dynamic, varying greatly with localities and seasons [ 77 , 78 , 79 ]. These variations of microbiota composition within field mosquitoes may partly explain the variability in infection levels in the field [ 80 ].
Mosquito microbiota has great potential for impeding the transmission of malaria by altering vectorial capacity [ 81 ]. Also, the microbiota is capable of influencing the biology of the host such as altering its immunity, nutrition, digestion, vectorial competence, reproduction, and insecticide resistance [ 82 , 83 , 84 , 85 , 86 , 87 ]. With the growing concerns about the rapid spread of insecticide resistance in Anopheles mosquitoes, some studies have explored the functions of the mosquito's gut microbial communities in the development of resistance. For example, distinct microbita populations were found associated with organochlorine resistance in An. arabiensis [ 86 ] and Anopheles albimanus [ 88 ]. Similarly, an association between specific microbiota and intense pyrethroid resistance was reported in An. gambiae [ 89 ] and Anopheles stephensi [ 90 ], suggesting a microbiota-mediated insecticide resistance mechanism. Dieme et al. [ 91 ] suggested that changes in the feeding behaviour of insecticide resistant vectors may lead to higher microbial diversity. This diversity could modify the repertoire of protective bacteria against pathogen infections and/or that of their enhancers, with consequences on the vectorial competence [ 9 , 92 ]. Recently, Bassene et al. [ 93 ] showed that, in the species An. gambiae and An. funestus , the microbiota was signifanctly different between P. falciparum -infected and non-infected samples, although the resistance status of these mosquitoes was not evaluated. More refined studies are needed to characterize the microbial communities harboured by the insecticide resistant malaria vectors. Also the contribution of microbiota against other factors to the vectorial competence of insecticide resistant malaria vectors remains to be investigated.
Impact of insecticide resistance on the longevity of malaria vectors
Mosquito longevity is a determinant factor for parasite maturation and could influences malaria transmission [ 94 , 95 ]. In fact, the extrinsic incubation of Plasmodium in its hematophageous host is about 11–14 days. Therefore, only mosquitoes whose lifespan is long enough could allow the complete development of the malaria parasite to the sporozoite infective stage and participate in the transmission of the disease. With the emergence and spread of insecticide resistance [ 7 ], many investigations were undertaken to gain knowledge of the effect of this phenomenon on the vectors’ longevity and so on its potential epidemiological impact.
So far, studies on the impact of insecticide resistance on malaria vectors longevity have focused on four species: An. gambiae, An. arabiensis, An. coluzzii and An. funestus . Globally, the findings revealed a pleitropic effect of insecticide resistance on mosquito lifespan [ 33 , 96 , 97 , 98 , 99 , 100 , 101 , 102 , 103 , 104 , 105 , 106 , 107 , 108 ] (Table 2 ). The majority of studies (10/14) which used laboratory strains showed that pyrethroid resistant An. funestus and An. gambiae live longer than susceptible ones [ 100 , 106 ]. Of the studies including field strains, a longer life span was reported in organochlorine and pyrethroid resistant An. funestus strains [ 104 , 105 ]. In contrast, a shorter life span was observed in An. gambiae strain resistant to organochlorine. It was reported that pre-exposure to insecticide in a manner micmicing field exposure to insecticides, affects the longevity of insecticide resistant An. gambiae strain [ 97 ], and that delayed mortality observed in the vectors may be dependent on resistance intensity [ 98 ]. This later observation indicates that findings obtained with laboratory colonies are to be taken with caution given that they may not reflect exactly what is oberved on the field [ 26 ]. Nevertheless, such studies remain important as they contribute to the understanding of the potential mechanisms affecting the vectors’ longevity [ 109 , 110 ], notably resource-based trade-off and oxidative stress.
Resource based trade-off is an evolutionary ecology concept that states that when environmental constraints lead to the augmentation of resources to one biological trait, other traits will have their energy budget reduced [ 111 ]. Accordingly, when mosquitoes adopt the detoxification mechanism to prevent the effect of insecticide, an increased production of detoxifying enzymes follows and is maintained by the additional resources deployed for the function. Otali et al. [ 100 ] have demonstrated that metabolism and longevity of insecticide resistant An. gambiae are lower than that of the susceptible strain. Moreover, they showed that the resistant strain has higher Reactive Oxygen Species (ROS), which are factors determining oxydative stress. In fact, the ROS are multifunctional molecules produced by cells of all organisms during normal metabolism [ 112 , 113 ]. They have been pointed out as key aging factor in other organisms including Anopheles [ 114 ]. Therefore, mosquitoes that develop the capacity to cope with oxydative stress are likely to live longer. Oliver and Brooke [ 103 ] in an experiment evaluating the effect of oxidative stress on the longevity of both An. arabiensis and An. funestus bearing respectively kdr and Cytochrome P450 mechanisms demonstrated that these species live longer, and that Cytochrome P450 activity seems more protective against oxydative stress.
Rivero et al. [ 26 ] proposed the potential effect of different detoxifying enzymes on vector longevity. For example, Glutathion S-Transferase is considered to protect against oxydative stress. Confirming this point, a longer lifespan implicating Glutathion S-Tranferase in An. funestus was revealed with and without exposure to insecticide [ 104 , 105 ]. In contrast, monoxygenase, known to be associated with an increase in oxydative stress has not led, as expected, to reduced longevity in An. funestus [ 108 ]. More studies using field populations and micmicing field conditions are necessary to ascertain the full impact of insecticide resistance on longevity of the malaria vector.
The need for a comprehensive understanding of the impact of insecticide resistance on malaria vector competence is unquestionable. The current state of knowledge is not only insufficient but also contradictory to draw a definitive conclusion. A tendency nevertheless emerges from findings that insecticide resistance may increases the infectivity of malaria vectors to Plasmodium , thus their vector competence. This is possibly due to changes in the expression of some genes notably those involved in blood-feeding and the immunity. Additionally, microbiota communities vary in the resistant mosquitoes as compared to the susceptible counterparts. The actual effect of these changes in the course of infection and their impact on the infectivity of malaria vectors to P. falciparum is still to be investigated. Finally, the longevity of the vectors is not always affected by insecticide resistance mechanisms. It is worth noting that, studies using vectors displaying metabolic resistance were under-represented because molecular markers to diagnose this character were developped only recently, especially in An. funestus . Malaria vectors that bear metabolic resistant mechanism are, on an ecological immunology point of view, expected to have a number of biological functions impaired, including immunity. If established, this situation may cause them to become less refractory to Plasmodium infection. Taking advantage of recent advances in the genomics, transcriptomics and molecular characterization of insecticide resistance, more refined studies can now be undertaken to fill knowledge gaps regarding the effect of insectide resistance on key determinants of vectorial competence and subsequently predict changes in the epidemiology of malaria in a context of insecticide resistance escalation.
Availability of data and materials
Not applicable.
Abbreviations
Dichloro-diphenyl-trichloroethane
Glutathione S-transferases
Knock down resistance
Lipopolysacharide
Phenoloxidase
Voltage gate sodium channel
WHO. A global brief on vector-borne diseases. Geneva, World Health Organization; 2014.
Gelband H, Panosian CB, Arrow KJ. Saving lives, buying time: economics of malaria drugs in an age of resistance. 2004.
WHO. World malaria report 2021. Geneva, World Health Organization; 2021.
Phillips M, Burrows J, Manyando C. Malaria. Nat Rev Dis Primers. 2017;3:17050.
Article Google Scholar
Cox FE. History of the discovery of the malaria parasites and their vectors. Parasit Vectors. 2010;3:5.
Sinka ME, Bangs MJ, Manguin S, Rubio-Palis Y, Chareonviriyaphap T, Coetzee M, et al. A global map of dominant malaria vectors. Parasit Vectors. 2012;5:69.
Riveron JM, Tchouakui M, Mugenzi L, Menze BD, Chiang M-C, Wondji CS. Insecticide resistance in malaria vectors: an update at a global scale. In Towards malaria elimination-a leap forward. IntechOpen; 2018.
Elliott R, Ramakrishna V. Insecticide resistance in Anopheles gambiae Giles. Nature. 1956;177:532–3.
Article CAS Google Scholar
Sheldon BC, Verhulst S. Ecological immunology: costly parasite defences and trade-offs in evolutionary ecology. Trends Ecol Evol. 1996;11:317–21.
Barnes KG, Weedall GD, Ndula M, Irving H, Mzihalowa T, Hemingway J, et al. Genomic footprints of selective sweeps from metabolic resistance to pyrethroids in African malaria vectors are driven by scale up of insecticide-based vector control. PLoS Genet. 2017;13: e1006539.
Donnelly MJ, Corbel V, Weetman D, Wilding CS, Williamson MS, Black WC. Does kdr genotype predict insecticide-resistance phenotype in mosquitoes? Trends Parasitol. 2009;25:213–9.
Weill M, Lutfalla G, Mogensen K, Chandre F, Berthomieu A, Berticat C, et al. Insecticide resistance in mosquito vectors. Nature. 2003;423:136–7.
Djogbénou L, Labbé P, Chandre F, Pasteur N, Weill M. Ace-1 duplication in Anopheles gambiae : a challenge for malaria control. Malar J. 2009;8:70.
Hemingway J, Hawkes NJ, McCarroll L, Ranson H. The molecular basis of insecticide resistance in mosquitoes. Insect Biochem Mol Biol. 2004;34:653–65.
Riveron JM, Irving H, Ndula M, Barnes KG, Ibrahim SS, Paine MJ, et al. Directionally selected cytochrome P450 alleles are driving the spread of pyrethroid resistance in the major malaria vector Anopheles funestus . Proc Natl Acad Sci USA. 2013;110:252–7.
Riveron JM, Ibrahim SS, Chanda E, Mzilahowa T, Cuamba N, Irving H, et al. The highly polymorphic CYP6M7 cytochrome P450 gene partners with the directionally selected CYP6P9a and CYP6P9b genes to expand the pyrethroid resistance front in the malaria vector Anopheles funestus in Africa. BMC Genomics. 2014;15:817.
Riveron JM, Yunta C, Ibrahim SS, Djouaka R, Irving H, Menze BD, et al. A single mutation in the GSTe2 gene allows tracking of metabolically based insecticide resistance in a major malaria vector. Genome Biol. 2014;15:R27.
Vlachou D, Schlegelmilch T, Runn E, Mendes A, Kafatos FC. The developmental migration of Plasmodium in mosquitoes. Curr Opin Genet Dev. 2006;16:384–91.
Levashina EA. Immune responses in Anopheles gambiae . Insect Biochem Mol Biol. 2004;34:673–8.
Söderhäll K. Invertebrate immunity. Springer Science & Business Media; 2011.
Cohuet A, Harris C, Robert V, Fontenille D. Evolutionary forces on Anopheles : what makes a malaria vector? Trends Parasitol. 2010;26:130–6.
Beier JC. Malaria parasite development in mosquitoes. Annu Rev Entomol. 1998;43:519–43.
Holme P, Masuda N. The basic reproduction number as a predictor for epidemic outbreaks in temporal networks. PLoS ONE. 2015;10: e0120567.
Dietz K. The estimation of the basic reproduction number for infectious diseases. Stat Methods Med Res. 1993;2:23–41.
Smith DL, McKenzie FE, Snow RW, Hay SI. Revisiting the basic reproductive number for malaria and its implications for malaria control. PLoS Biol. 2007;5: e42.
Rivero A, Vézilier J, Weill M, Read AF, Gandon S. Insecticide control of vector-borne diseases: when is insecticide resistance a problem? PLoS Pathog. 2010;6: e1001000.
Kabula B, Tungu P, Rippon EJ, Steen K, Kisinza W, Magesa S, et al. A significant association between deltamethrin resistance, Plasmodium falciparum infection and the Vgsc-1014S resistance mutation in Anopheles gambiae highlights the epidemiological importance of resistance markers. Malar J. 2016;15:289.
Tchouakui M, Chiang MC, Ndo C, Kuicheu CK, Amvongo-Adjia N, Wondji MJ, et al. A marker of glutathione S-transferase-mediated resistance to insecticides is associated with higher Plasmodium infection in the African malaria vector Anopheles funestus . Sci Rep. 2019;9:5772.
Collins E, Vaselli NM, Sylla M, Beavogui AH, Orsborne J, Lawrence G, et al. The relationship between insecticide resistance, mosquito age and malaria prevalence in Anopheles gambiae s.l. from Guinea. Sci Rep. 2019;9:8846.
Ndiath MO, Cailleau A, Diedhiou SM, Gaye A, Boudin C, Richard V, et al. Effects of the kdr resistance mutation on the susceptibility of wild Anopheles gambiae populations to Plasmodium falciparum : a hindrance for vector control. Malar J. 2014;3:340.
Ndo C, Kopya E, Irving H, Wondji C. Exploring the impact of glutathione S-transferase (GST)-based metabolic resistance to insecticide on vector competence of Anopheles funestus for Plasmodium falciparum . Wellcome Open Res. 2019;4:52.
Alout H, Ndam NT, Sandeu MM, Djégbe I, Chandre F, Dabiré RK, et al. Insecticide resistance alleles affect vector competence of Anopheles gambiae s.s. for Plasmodium falciparum field isolates. PLoS ONE. 2013;8:e63849.
Alout H, Dabiré RK, Djogbénou LS, Abate L, Corbel V, Chandre F, et al. Interactive cost of Plasmodium infection and insecticide resistance in the malaria vector Anopheles gambiae . Sci Rep. 2016;6:29755.
Alout H, Djègbè I, Chandre F, Djogbénou LS, Dabiré RK, Corbel V, et al. Insecticide exposure impacts vector-parasite interactions in insecticide-resistant malaria vectors. Proc Biol Sci. 2014;281:20140389.
Google Scholar
Kristan M, Abeku TA, Lines J. Effect of environmental variables and kdr resistance genotype on survival probability and infection rates in Anopheles gambiae (s.s.). Parasit Vectors. 2018;11:560.
Lo TM, Coetzee M. Marked biological differences between insecticide resistant and susceptible strains of Anopheles funestus infected with the murine parasite Plasmodium berghei . Parasit Vectors. 2013;6:184.
Ribeiro JM, Francischetti IM. Role of arthropod saliva in blood feeding: sialome and post-sialome perspectives. Annu Rev Entomol. 2003;48:73–88.
Calvo E, Dao A, Pham VM, Ribeiro JM. An insight into the sialome of Anopheles funestus reveals an emerging pattern in anopheline salivary protein families. Insect Biochem Mol Biol. 2007;37:164–75.
Das S, Radtke A, Choi YJ, Mendes AM, Valenzuela JG, Dimopoulos G. Transcriptomic and functional analysis of the Anopheles gambiae salivary gland in relation to blood feeding. BMC Genomics. 2010;11:566.
Elanga-Ndille E, Nouage L, Binyang A, Assatse T, Tene-Fossog B, Tchouakui M, et al. Overexpression of two members of D7 salivary genes family is associated with pyrethroid resistance in the malaria vector Anopheles funestus s.s. but not in Anopheles gambiae in Cameroon. Genes (Basel). 2019;10:211.
Vijay S, Rawal R, Kadian K, Raghavendra K, Sharma A. Annotated differentially expressed salivary proteins of susceptible and insecticide-resistant mosquitoes of Anopheles stephensi . PLoS ONE. 2015;10: e0119666.
Ghosh AK, Devenport M, Jethwaney D, Kalume DE, Pandey A, Anderson VE, et al. Malaria parasite invasion of the mosquito salivary gland requires interaction between the Plasmodium TRAP and the Anopheles saglin proteins. PLoS Pathog. 2009;5: e1000265.
Cornelie S, Rossignol M, Seveno M, Demettre E, Mouchet F, Djègbè I, et al. Salivary gland proteome analysis reveals modulation of anopheline unique proteins in insensitive acetylcholinesterase resistant Anopheles gambiae mosquitoes. PLoS ONE. 2014;9: e103816.
Mueller AK, Kohlhepp F, Hammerschmidt C, Michel K. Invasion of mosquito salivary glands by malaria parasites: prerequisites and defense strategies. Int J Parasitol. 2010;40:1229–35.
Wells MB, Andrew DJ. Anopheles salivary gland architecture shapes Plasmodium sporozoite availability for transmission. MBio. 2019;10:e01238-e1319.
O’Brochta DA, Alford R, Harrell R, Aluvihare C, Eappen AG, Li T, et al. Is Saglin a mosquito salivary gland receptor for Plasmodium falciparum ? Malar J. 2019;18:2.
Calvo E, Mans BJ, Andersen JF, Ribeiro JM. Function and evolution of a mosquito salivary protein family. J Biol Chem. 2006;281:1935–42.
Francischetti IM, Valenzuela JG, Pham VM, Garfield MK, Ribeiro JM. Toward a catalog for the transcripts and proteins (sialome) from the salivary gland of the malaria vector Anopheles gambiae . J Exp Biol. 2002;205:2429–51.
Isaacs AT, Mawejje HD, Tomlinson S, Rigden DJ, Donnelly MJ. Genome-wide transcriptional analyses in Anopheles mosquitoes reveal an unexpected association between salivary gland gene expression and insecticide resistance. BMC Genomics. 2018;19:225.
Beerntsen BT, James AA, Christensen BM. Genetics of mosquito vector competence. Microbiol Mol Biol Rev. 2000;64:115–37.
Djegbe I, Cornelie S, Rossignol M, Demettre E, Seveno M, Remoue F, Corbel V. Differential expression of salivary proteins between susceptible and insecticide-resistant mosquitoes of Culex quinquefasciatus . PLoS ONE. 2011;6: e17496.
Mano C, Jariyapan N, Sor-Suwan S, Roytrakul S, Kittisenachai S, Tippawangkosol P, et al. Protein expression in female salivary glands of pyrethroid-susceptible and resistant strains of Aedes aegypti mosquitoes. Parasit Vectors. 2019;12:111.
Rosinski-Chupin I, Briolay J, Brouilly P, Perrot S, Gomez SM, Chertemps T, et al. SAGE analysis of mosquito salivary gland transcriptomes during Plasmodium invasion. Cell Microbiol. 2007;9:708–24.
Sor-suwan S, Jariyapan N, Roytrakul S, Paemanee A, Phumee A, Phattanawiboon B, et al. Identification of salivary gland proteins depleted after blood feeding in the malaria vector Anopheles campestris -like mosquitoes (Diptera: Culicidae). PLoS ONE. 2014;9: e90809.
Cázares-Raga FE, Chávez-Munguía B, González-Calixto C, Ochoa-Franco AP, Gawinowicz MA, Rodríguez MH, Hernández-Hernández FC. Morphological and proteomic characterization of midgut of the malaria vector Anopheles albimanus at early time after a blood feeding. J Proteomics. 2014;111:100–12.
Villalon JM, Ghosh A, Jacobs-Lorena M. The peritrophic matrix limits the rate of digestion in adult Anopheles stephensi and Aedes aegypti mosquitoes. J Insect Physiol. 2003;49:891–5.
Billingsley PF. Blood digestion in the mosquito, Anopheles stephensi Liston (Diptera: Culicidae): partial characterization and post-feeding activity of midgut aminopeptidases. Arch Insect Biochem Physiol. 1990;15:149–63.
Dana AN, Hong YS, Kern MK, Hillenmeyer ME, Harker BW, Lobo NF, et al. Gene expression patterns associated with blood-feeding in the malaria mosquito Anopheles gambiae . BMC Genomics. 2005;6:5.
Billingsley PF, Hecker H. Blood digestion in the mosquito, Anopheles stephensi Liston (Diptera: Culicidae): activity and distribution of trypsin, aminopeptidase, and alpha-glucosidase in the midgut. J Med Entomol. 1991;28:865–71.
Ribeiro JM. A catalogue of Anopheles gambiae transcripts significantly more or less expressed following a blood meal. Insect Biochem Mol Biol. 2003;33:865–82.
Vijay S, Rawal R, Kadian K, Singh J, Adak T, Sharma A. Proteome-wide analysis of Anopheles culicifacies mosquito midgut: new insights into the mechanism of refractoriness. BMC Genomics. 2018;19:337.
Mitri C, Markianos K, Guelbeogo WM, Bischoff E, Gneme A, Eiglmeier K, et al. The kdr-bearing haplotype and susceptibility to Plasmodium falciparum in Anopheles gambiae : genetic correlation and functional testing. Malar J. 2015;14:391.
Vontas J, Blass C, Koutsos AC, David JP, Kafatos FC, Louis C, et al. Gene expression in insecticide resistant and susceptible Anopheles gambiae strains constitutively or after insecticide exposure. Insect Mol Biol. 2005;14:509–21.
Ingham VA, Brown F, Ranson H. Transcriptomic analysis reveals pronounced changes in gene expression due to sub-lethal pyrethroid exposure and ageing in insecticide resistance Anopheles coluzzii . BMC Genomics. 2021;22:337.
Hamer GL, Kitron UD, Brawn JD, Loss SR, Ruiz MO, Goldberg TL, et al. Culex pipiens (Diptera: Culicidae): a bridge vector of West Nile virus to humans. J Med Entomol. 2008;45:125–8.
Morchón R, Bargues MD, Latorre JM, Melero-Alcíbar R, Pou-Barreto C, Mas-Coma S, et al. Haplotype H1 of Culex pipiens implicated as natural vector of Dirofilaria immitis in an endemic area of Western Spain. Vector Borne Zoonotic Dis. 2007;7:653–8.
Kimura M, Darbro JM, Harrington LC. Avian malaria parasites share congeneric mosquito vectors. J Parasitol. 2010;96:144–51.
Vézilier J, Nicot A, Lorgeril J, Gandon S, Rivero A. The impact of insecticide resistance on Culex pipiens immunity. Evol Appl. 2013;6:497–509.
Molina-Cruz A, DeJong RJ, Charles B, Gupta L, Kumar S, Jaramillo-Gutierrez G, et al. Reactive oxygen species modulate Anopheles gambiae immunity against bacteria and Plasmodium . J Biol Chem. 2008;283:3217–23.
Kumar S, Christophides GK, Cantera R, Charles B, Han YS, Meister S, et al. The role of reactive oxygen species on Plasmodium melanotic encapsulation in Anopheles gambiae . Proc Natl Acad Sci USA. 2003;100:14139–44.
James RR, Xu J. Mechanisms by which pesticides affect insect immunity. J Invertebr Pathol. 2012;109:175–82.
Hillyer JF, Strand MR. Mosquito hemocyte-mediated immune responses. Curr Opin Insect Sci. 2014;3:14–21.
Lavine MD, Strand MR. Insect hemocytes and their role in immunity. Insect Biochem Mol Biol. 2002;32:1295–309.
Cornet S, Gandon S, Rivero A. Patterns of phenoloxidase activity in insecticide resistant and susceptible mosquitoes differ between laboratory-selected and wild-caught individuals. Parasit Vectors. 2013;6:315.
Wang Y, Gilbreath TM 3rd, Kukutla P, Yan G, Xu J. Dynamic gut microbiome across life history of the malaria mosquito Anopheles gambiae in Kenya. PLoS ONE. 2011;6: e24767.
Gimonneau G, Tchioffo MT, Abate L, Boissière A, Awono-Ambéné PH, Nsango SE, et al. Composition of Anopheles coluzzii and Anopheles gambiae microbiota from larval to adult stages. Infect Genet Evol. 2014;28:715–24.
Akorli J, Gendrin M, Pels NA, Yeboah-Manu D, Christophides GK, Wilson MD. Seasonality and locality affect the diversity of Anopheles gambiae and Anopheles coluzzii midgut microbiota from Ghana. PLoS ONE. 2016;11: e0157529.
Krajacich BJ, Huestis DL, Dao A, Yaro AS, Diallo M, Krishna A, Xu J, Lehmann T. Investigation of the seasonal microbiome of Anopheles coluzzii mosquitoes in Mali. PLoS ONE. 2018;13: e0194899.
Sandeu MM, Maffo CGT, Dada N, Njiokou F, Hughes GL, Wondji CS. Seasonal variation of microbiota composition in Anopheles gambiae and Anopheles coluzzii in two different eco-geographical localities in Cameroon. Med Vet Entomol. 2022;36:269–82.
Rosenberg R. Malaria: some considerations regarding parasite productivity. Trends Parasitol. 2008;24:487–91.
Cansado-Utrilla C, Zhao SY, McCall PJ, Coon KL, Hughes GL. The microbiome and mosquito vectorial capacity: rich potential for discovery and translation. Microbiome. 2021;9:111.
Rodgers FH, Gendrin M, Wyer CAS, Christophides GK. Microbiota-induced peritrophic matrix regulates midgut homeostasis and prevents systemic infection of malaria vector mosquitoes. PLoS Pathog. 2017;13: e1006391.
Song X, Wang M, Dong L, Zhu H, Wang J. PGRP-LD mediates A. stephensi vector competency by regulating homeostasis of microbiota-induced peritrophic matrix synthesis. PLoS Pathog. 2018;14:e1006899.
Romoli O, Gendrin M. The tripartite interactions between the mosquito, its microbiota and Plasmodium . Parasit Vectors. 2018;11:200.
Barletta ABF, Trisnadi N, Ramirez JL, Barillas-Mury C. Mosquito midgut prostaglandin release establishes systemic immune priming. iScience. 2019;19:54–62.
Barnard K, Jeanrenaud A, Brooke BD, Oliver SV. The contribution of gut bacteria to insecticide resistance and the life histories of the major malaria vector Anopheles arabiensis (Diptera: Culicidae). Sci Rep. 2019;9:9117.
Martinson VG, Strand MR. Diet-microbiota interactions alter mosquito development. Front Microbiol. 2021;12: 650743.
Dada N, Sheth M, Liebman K, Pinto J, Lenhart A. Whole metagenome sequencing reveals links between mosquito microbiota and insecticide resistance in malaria vectors. Sci Rep. 2018;8:2084.
Omoke D, Kipsum M, Otieno S, Esalimba E, Sheth M, Lenhart A, et al. Western Kenyan Anopheles gambiae showing intense permethrin resistance harbour distinct microbiota. Malar J. 2021;20:77.
Soltani A, Vatandoost H, Oshaghi MA, Enayati AA, Chavshin AR. The role of midgut symbiotic bacteria in resistance of Anopheles stephensi (Diptera: Culicidae) to organophosphate insecticides. Pathog Glob Health. 2017;111:289–96.
Dieme C, Rotureau B, Mitri C. Microbial pre-exposure and vectorial competence of Anopheles mosquitoes. Front Cell Infect Microbiol. 2017;7:508.
Gabrieli P, Caccia S, Varotto-Boccazzi I, Arnoldi I, Barbieri G, Comandatore F, et al. Mosquito trilogy: microbiota, immunity and pathogens, and their implications for the control of disease transmission. Front Microbiol. 2021;12: 630438.
Bassene H, Niang EHA, Fenollar F, Dipankar B, Doucouré S, Ali E, et al. 6S Metagenomic comparison of Plasmodium falciparum -infected and noninfected Anopheles gambiae and Anopheles funestus microbiota from Senegal. Am J Trop Med Hyg. 2018;99:1489–98.
Garrett-Jones C, Shidrawi GR. Malaria vectorial capacity of a population of Anopheles gambiae : an exercise in epidemiological entomology. Bull World Health Organ. 1969;40:531–45.
CAS Google Scholar
Ferguson HM, Maire N, Takken W, Lyimo IN, Briët O, Lindsay SW, Smith TA. Selection of mosquito life-histories: a hidden weapon against malaria? Malar J. 2012;11:106.
Nkahe DL, Kopya E, Djiappi-Tchamen B, Toussile W, Sonhafouo-Chiana N, Kekeunou S, et al. Fitness cost of insecticide resistance on the life-traits of a Anopheles coluzzii population from the city of Yaoundé, Cameroon. Wellcome Open Res. 2020;5:171.
Msangi G, Olotu MI, Mahande AM, Philbert A, Kweka EJ. The impact of insecticide pre-exposure on longevity, feeding succession, and egg batch size of wild Anopheles gambiae s.l. J Trop Med. 2020;2020:8017187.
Hughes A, Lissenden N, Viana M, Toé KH, Ranson H. Anopheles gambiae populations from Burkina Faso show minimal delayed mortality after exposure to insecticide-treated nets. Parasit Vectors. 2020;13:17.
Viana M, Hughes A, Matthiopoulos J, Ranson H, Ferguson HM. Delayed mortality effects cut the malaria transmission potential of insecticide-resistant mosquitoes. Proc Natl Acad Sci USA. 2016;113:8975–80.
Otali D, Novak RJ, Wan W, Bu S, Moellering DR, De Luca M. Increased production of mitochondrial reactive oxygen species and reduced adult life span in an insecticide-resistant strain of Anopheles gambiae . Bull Entomol Res. 2014;104:323–33.
Oliver SV, Brooke BD. The effect of elevated temperatures on the life history and insecticide resistance phenotype of the major malaria vector Anopheles arabiensis (Diptera: Culicidae). Malar J. 2017;16:73.
Oliver SV, Brooke BD. The effect of multiple blood-feeding on the longevity and insecticide resistant phenotype in the major malaria vector Anopheles arabiensis (Diptera: Culicidae). Parasit Vectors. 2014;7:390.
Oliver SV, Brooke BD. The role of oxidative stress in the longevity and insecticide resistance phenotype of the major malaria vectors Anopheles arabiensis and Anopheles funestus . PLoS ONE. 2016;11: e0151049.
Tchakounte A, Tchouakui M, Mu-Chun C, Tchapga W, Kopia E, Soh PT, et al. Exposure to the insecticide-treated bednet PermaNet 2.0 reduces the longevity of the wild African malaria vector Anopheles funestus but GSTe2-resistant mosquitoes live longer. PLoS ONE. 2019;14:e0213949.
Tchouakui M, Riveron JM, Djonabaye D, Tchapga W, Irving H, Soh Takam P, et al. Fitness Costs of the glutathione S-transferase epsilon 2 (L119F-GSTe2) mediated metabolic resistance to insecticides in the major African malaria vector Anopheles funestus . Genes (Basel). 2018;9:645.
Okoye PN, Brooke BD, Hunt RH, Coetzee M. Relative developmental and reproductive fitness associated with pyrethroid resistance in the major southern African malaria vector, Anopheles funestus . Bull Entomol Res. 2007;97:599–605.
Tchouakui M, Riveron Miranda J, Mugenzi LMJ, Djonabaye D, Wondji MJ, Tchoupo M, et al. Cytochrome P450 metabolic resistance (CYP6P9a) to pyrethroids imposes a fitness cost in the major African malaria vector Anopheles funestus . Heredity (Edinb). 2020;124:621–32.
Tchouakui M, Mugenzi LMJ, Wondji MJ, Tchoupo M, Njiokou F, Wondji CS. Combined over-expression of two cytochrome P450 genes exacerbates the fitness cost of pyrethroid resistance in the major African malaria vector Anopheles funestus . Pestic Biochem Physiol. 2021;173: 104772.
Remick D. Measuring the costs of reproduction. Trends Ecol Evol. 1992;7:42–5.
Kirkwood TB, Rose MR. Evolution of senescence: late survival sacrificed for reproduction. Philos Trans R Soc Lond B Biol Sci. 1991;332:15–24.
Garland T Jr. Trade-offs. Curr Biol. 2014;24:R60–1.
Rada B, Leto TL. Oxidative innate immune defenses by Nox/Duox family NADPH oxidases. Contrib Microbiol. 2008;15:164–87.
Sumimoto H. Structure, regulation and evolution of Nox-family NADPH oxidases that produce reactive oxygen species. FEBS J. 2008;275:3249–77.
Monaghan P, Metcalfe NB, Torres R. Oxidative stress as a mediator of life history trade-offs: mechanisms, measurements and interpretation. Ecol Lett. 2009;12:75–92.
Download references
The authors acknowledge the financial support from the Wellcome Trust, UK, through the International Intermediate Fellowship (220720/Z/20/Z) granted to Cyrille NDO.
Author information
Authors and affiliations.
Department of Parasitology and Microbiology, Centre for Research in Infectious Diseases, P.O. Box 13591, Yaoundé, Cameroon
Pierre Fongho Suh, Darus Tagne, Charles Wondji & Cyrille Ndo
Faculty of Sciences, University of Yaoundé I, P.O. Box 837, Yaoundé, Cameroon
Pierre Fongho Suh
Department of Medical Entomology, Centre for Research in Infectious Diseases, P.O. Box 13591, Yaoundé, Cameroon
Emmanuel Elanga-Ndille, Magellan Tchouakui & Maurice Marcel Sandeu
Department of Microbiology and Infectious Diseases, School of Veterinary Medicine and Sciences, University of Ngaoundéré, P.O. Box 454, Ngaoundéré, Cameroon
Maurice Marcel Sandeu
Faculty of Sciences, University of Douala, P.O. Box 24157, Douala, Cameroon
Darus Tagne
Vector Biology Department, Liverpool School of Tropical Medicine, Pembroke Place, Liverpool, L3 5QA, UK
Charles Wondji
Department of Biological Sciences, Faculty of Medicine and Pharmaceutical Sciences, University of Douala, P.O. Box 24157, Douala, Cameroon
Cyrille Ndo
You can also search for this author in PubMed Google Scholar
Contributions
PFS, EEN, MT, MMS, CN wrote the manuscript. TD and PFS prepared the figures and tables. CN and CW acquiered the funding. All the authors revised the manuscript. All authors read and approved the final manuscript.
Corresponding author
Correspondence to Cyrille Ndo .
Ethics declarations
Ethics approval and consent to participate, consent for publication, competing interests.
The authors declare no competing interests.
Additional information
Publisher's note.
Springer Nature remains neutral with regard to jurisdictional claims in published maps and institutional affiliations.
Rights and permissions
Open Access This article is licensed under a Creative Commons Attribution 4.0 International License, which permits use, sharing, adaptation, distribution and reproduction in any medium or format, as long as you give appropriate credit to the original author(s) and the source, provide a link to the Creative Commons licence, and indicate if changes were made. The images or other third party material in this article are included in the article's Creative Commons licence, unless indicated otherwise in a credit line to the material. If material is not included in the article's Creative Commons licence and your intended use is not permitted by statutory regulation or exceeds the permitted use, you will need to obtain permission directly from the copyright holder. To view a copy of this licence, visit http://creativecommons.org/licenses/by/4.0/ . The Creative Commons Public Domain Dedication waiver ( http://creativecommons.org/publicdomain/zero/1.0/ ) applies to the data made available in this article, unless otherwise stated in a credit line to the data.
Reprints and permissions
About this article
Cite this article.
Suh, P.F., Elanga-Ndille, E., Tchouakui, M. et al. Impact of insecticide resistance on malaria vector competence: a literature review. Malar J 22 , 19 (2023). https://doi.org/10.1186/s12936-023-04444-2
Download citation
Received : 23 August 2022
Accepted : 04 January 2023
Published : 17 January 2023
DOI : https://doi.org/10.1186/s12936-023-04444-2

Share this article
Anyone you share the following link with will be able to read this content:
Sorry, a shareable link is not currently available for this article.
Provided by the Springer Nature SharedIt content-sharing initiative
- Insecticide resistance
- Vector competence
Malaria Journal
ISSN: 1475-2875
- Submission enquiries: [email protected]
Characterization of urinary metabolites associated with malaria infection using infra-red spectroscopy and liquid chromatography–mass spectrometry in South Western Uganda
- Open access
- Published: 28 June 2024
- Volume 6 , article number 356 , ( 2024 )
Cite this article
You have full access to this open access article
- Grace Birungi ORCID: orcid.org/0000-0002-8520-017X 1 ,
- Joan Beryl Achar 1 &
- Denis Byamugisha 1
Early malaria diagnosis improves outcomes during malaria treatment; routine diagnostic techniques rely on blood samples obtained invasively. Therefore, this study used infra-red (IR) spectroscopy coupled with Principle Component Analysis (PCA) to study the urinary profile of malaria patients and that of controls aimed at understanding metabolite perturbation during malaria infection so as to contribute towards development of non-invasive malaria diagnosis methods. Freeze dried human urine samples form malaria infected individuals (cases) and controls were screened in the IR region of 4000 cm −1 to 600 cm −1 and overall spectral differences were observed at wave numbers 1618 cm −1 , 1679 cm −1 (amino acids). Peaks at 3030 cm −1 (NH 4 + ) and 940 cm −1 (O–H of carboxylic acids) showed high absorbance in patients compared to controls. Liquid-chromatography–mass spectrometry (LC–MS/MS) was used to quantify amino acids in the urine samples and the results indicated a significant increase of amino acid cystine (P = 0.012). Lysine and tyrosine also increased in patients compared to controls. The use of IR-PCA differentiated clusters of urine samples from patients with malaria from control and the demonstrated amino acid perturbation is consistent with malaria infection. This data provides baseline information for application in development of a non-invasive diagnostic tests for malaria.
Article Highlights
Infrared (IR) spectroscopy and Principle Component Analysis (PCA) were used to differentiate urine of malaria patients from controls.
LC–MS/MS was used to determine creatinine, tyrosine, cystine, lysine and histidine in urine from malaria patients and controls
The average concentration of cystine in patients and controls urine differed significantly.
Avoid common mistakes on your manuscript.
1 Introduction
Malaria is a disastrous mosquito-borne disease caused by protozoan parasites of the genus Plasmodium including: Plasmodium falciparum , Plasmodium vivax , Plasmodium malariae , Plasmodium ovale and Plasmodium knowlesi [ 1 ] . Plasmodium falciparum is the deadliest [ 2 ] and most prevalent on the African continent where malaria still exacts a large disease burden [ 3 ]. For example in 2022, out of the 249 million malaria cases in 85 malaria endemic countries globally, 93.6% of cases and 95.4% of deaths were from World Health Organization (WHO) African region, with children under the age of 5 years accounting for 78.1% of all malaria deaths in that year [ 4 ]. Preventive measures including seasonal malaria chemoprevention [ 5 ] and personal protection against mosquito bites [ 6 , 7 ] have been used although malaria incidence is still high. In Uganda, the WHO reported an estimated 12.7 million malaria cases and over 17,556 deaths in the year 2022 [ 4 ] showing that malaria is a significant threat to health, therefore efforts should be made towards malaria control and management. Early diagnosis and treatment of malaria contributes to reducing transmission and prevents deaths [ 8 , 9 ], therefore it is key.
The gold standard for malaria diagnosis is microscopy where a blood sample is drawn from a patient and examined for presence of plasmodium. Microscopic examination of Giemsa-stained blood thick smears (1.0 × 10 6 RBCs) or thin blood smears (1.25 × 10 5 RBCs) is used to confirm presence of plasmodium based on the morphological features after examination with 100X oil immersion objective [ 10 , 11 ]. The accuracy of diagnosis can be challenging even to expert microscopists; therefore, other techniques have been developed. For example, Rapid Diagnostic Tests (RDTs) which rely on the detection of P. falciparum histidine-rich protein 2 (PfHRP2) or its structural homolog PfHRP3, are commonly used today although they can also report false-positive and false-negatives [ 6 ].
The potential of using urine and saliva specimens for malaria diagnosis was investigated in Ghana where blood, saliva and urine were tested for malaria antigens PfHRP2 and parasite lactate dehydrogenase (pLDH). While the sensitivities of urine and saliva were low (35.2% and 57.0% respectively) the use of these non-blood specimens demonstrated a potential for use as non-invasive samples for malaria diagnosis although this approach was only useful in severe parasitemia > 60,000 parasites/µL [ 12 ]. Other diagnostic methods which use DNA probes, polymerase chain reaction (PCR) and fluorescent staining, have been reported [ 10 ] but require collection of capillary blood moreover molecular based methods are expensive and require more expertise [ 13 ].
Collection of a blood sample for diagnostic purposes is invasive and may expose both the health worker and patient to other infections arising from the manipulation of potentially infectious bodily fluids [ 14 ] therefore, non-invasive alternative diagnostic methods could be an added advantage. Non-invasive tests for malaria such as the detection of plasmodia parasite antigens or DNA in samples such as saliva, urine or buccal mucosa [ 12 , 13 ] and the use of skin volatiles as predictors of infection status [ 13 , 14 , 15 ] have been proposed. Most of these methods are still in their infancy, although the use of saliva- and urine-based tests is well advanced for diagnosis of other infections [ 13 ].
Severe Plasmodium falciparum infection results in alteration of microvasculature of various tissues such as liver, kidney and intestines due to isolation of parasitized red blood cells, leading to possible metabolic stress [ 16 ]. Late stages of malaria result in complications due to inflammatory immune responses and one or more conditions such as cerebral malaria, renal damage, liver damage, severe anaemia, acidosis and hypoglycaemia which may lead to death [ 17 ]. Manifestations of malaria are associated with drastic metabolic alterations in the host, therefore, assessing metabolites in body fluids and tissues can potentially provide information on metabolite changes during malaria infection [ 18 , 19 ].
Urine is an ideal bio-fluid for disease study because it is rich in metabolites, readily available, easily obtained, can be collected in large volumes and is mostly free from interfering proteins or lipids compared to other body fluids [ 20 , 21 , 22 ]. Urine has been used in metabolite profiling studies due to its low protein content and limited sample pre-treatment; moreover, it can be collected non-invasively and longitudinally [ 19 , 20 , 23 ]. Approximately 5661 metabolites have been documented in urine [ 22 ], associated with about 600 human conditions [ 23 ] including obesity, cancer, inflammation neurological disease, and other infectious diseases.
A large number of metabolite profiling reports rely on high end techniques such as LC–MS [ 11 , 19 , 24 ], yet these techniques are not readily available in low and developing economies, therefore, in this study, human urine was investigated for potential metabolite fingerprinting associated with plasmodium malaria infection using Infrared (IR) spectroscopy coupled with chemometry. Infrared spectroscopy of urine has shown potential application in non-invasive cancer diagnosis [ 25 , 26 , 27 ] and has also been used in the detection of malaria parasites in blood [ 28 , 29 ]. In this study, we explored the application of Infrared spectroscopy to distinguish between urine of malaria patients and controls in South Western Uganda in an attempt to determine molecular signatures associated with malaria infection. Identification of molecules perturbed during malaria infection can contribute towards development of non-invasive diagnostic kits or methods; hence liquid chromatography–mass spectrometry (LC–MS) was used to validate potential metabolite markers. The information obtained from this study provides baseline information for future development of a non-invasive technique for malaria diagnosis.
2 Materials and methods
2.1 study site.
The study was done in Mbarara City, located in the South Western region of Uganda. Mbarara City the second largest city after Kampala (Latitude of 0.6072° S longitude of 30.6545° E) and about 167 miles (270 km) southwest of Kampala as shown in Fig. 1 .
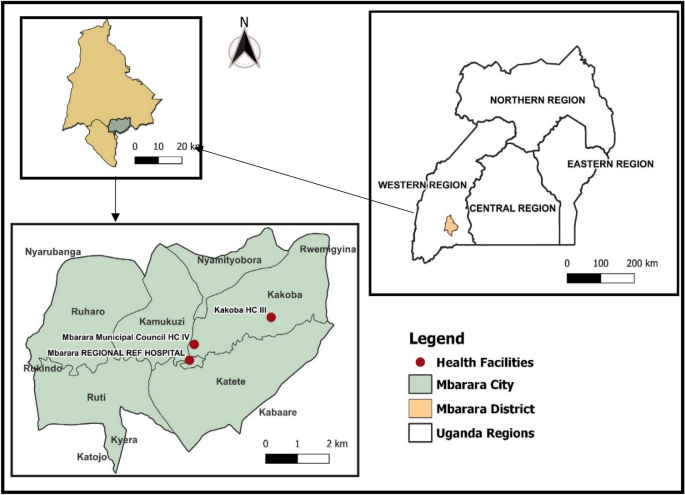
Map of Mbarara City showing the health facilities from which samples were collected
2.2 Study design
A mixed method design was used to collect qualitative and quantitative data. A case–control study design was used; urine samples were collected from malaria patients (cases), who were confirmed Plasmodium falciparum positive using blood film microscopy regardless of the level of parasitemia, before treatment. Controls were sex-matched healthy people with similar age to the cases and had negative test results for malaria. Participants in this study were children (3–5 years), adolescents (10–19) and adults (20–50 years). Only children who were toilet trained were selected in order to collect mid-stream urine which reduces the risk of bacterial contamination. All genders participated and a primary screening included a physician taking a brief history of the participants and a simple urinalysis. Inclusion criteria: Non-obese (BMI < 30 kg/m 2 ), non-pregnant or lactating for women, not diagnosed with a chronic or acute disease (apart from malaria), and not receiving any other drugs, and supplements. Exclusion criteria: Detection of: leucocyte, protein, glucose, bilirubin and nitrite, urine pH less than 5 or more than 7 and urinary system complaints [ 29 ]. Qualitative data including demographics data about patients was collected using a questionnaire.
2.3 Ethics approval and consent to participate
All experiments were performed in accordance with the Uganda National Guidelines for Research involving Humans as Research Participants [ 30 ]. Informed consent was provided by all the participants (parents/guardians in case of participants under the age of 18 years). Ethical clearance was obtained from Mbarara University Research and Ethics Committee (MUST-2022-590).
2.4 Sample collection, preparation and storage
Mid-stream urine (40 ml) was collected randomly [ 31 ] from 112 participants (41 patients and 71 controls) whose malaria status had been confirmed through microscopy diagnosis. The microscopy samples were assigned (+) for < 100 parasites/µl of blood (mild malaria), (++) for 100–9999 parasites/µl of blood (moderate malaria), and (+++) for ≥ 10,000 parasite/µl of blood (severe malaria) [ 32 ]. Urine samples were self-collected into clean urine containers in adults while children (3–5 years), collected samples with the help of the parent/guardian and the laboratory technologist and carried in a cool box; no preservatives were used. Simple urinalysis was done to check for any unwanted contaminants using reagent strips [ 11 ]. Centrifugation was done at 2000 rpm for 15 min using LC-3000 centrifuge to remove insoluble materials and cellular debris and samples were stored at − 20 °C awaiting freeze drying. Urine samples (30 ml) were freeze-dried using Benchtop Freeze Dryer to remove excess water by placing a sample which was previously frozen at − 80 °C under a vacuum at − 86− and 1 Pascal allowing direct change of the ice to vapour without passing through liquid phase for 18 h.
2.5 Sample analysis using infra-red
The freeze-dried sample (1 mg) was re-dissolved in acetonitrile (1 ml) and vortexed for 2 min. The reconstituted urine sample (5 µl) was dropped onto the diamond crystal surface in an Infrared Spectrophotometer (IR) (Buck 530) and scanned for 3 min in the range from 4000 cm −1 to 600 cm −1 after correction for background interference. The diamond crystal was cleaned with acetonitrile after running each sample [ 29 ].
2.6 Sample analysis using LC–MS
2.6.1 analytical method validation.
Amino acids standards (Glentham Life Sciences) were used for preparation of stock solutions by dissolving in LC–MS grade water containing 0.1% formic acid. Working standards solutions were made by diluting of stock solutions. Amino acids solution mixtures of 10, 20, 50,100, 200 and 500 ppb in milli-Q water containing 0.1% formic acid were run and calibration curves were obtained by plotting the peak area against the concentration of the standard analysed. Confirmation of the amino acids was performed based on multiple reaction monitoring (MRM) transitions of the parent and daughter ions for each analyte. Analytical method parameters are indicated in supplementary data.
2.6.2 Quality assurance and quality control procedures
The samples were collected in clean urine containers without any preservatives, prepared and stored in the freezer (− 20 °C) within 30 min of collection to ensure integrity. Freeze-drying of the samples was done to increase the stability of the analytes. The repeatability of the data and stability of the IR equipment were determined by running one sample six times followed by data analysis using Kruskal Wallis ANOVA. LC–MS/MS parameters were verified by determining repeatability, linearity, limit of detection (LOD) and limit of quantification (LOQ). Repeatability was done by analysing each standard six times at two different concentrations: 10 ppb and 500 ppb. Linearity was based on the calibration curves of amino acids mixture within the range of 10 to 500 ppb. The LC–MS/MS LODs and LOQs were defined as the amino acids concentrations required to produce signal to noise ratio of 3:1 and 10:1 respectively. All laboratory and clinical data were entered into the corresponding logbooks and transcribed into Excel worksheet (Windows 10, Microsoft Inc., and Richmond, Washington 2011) and analysed with Origin Pro version 2017 SR26.
2.6.3 Determination of amino acids in urine samples using LC–MS/MS
The freeze-dried urine sample (0.01 mg) was dissolved in 1mL milli-Q-water containing 0.1% formic acid, then filtered through a 0.22 µm syringe -adaptable filter into LC vials and later diluted for analysis. An Agilent 1100 series liquid chromatograph (LC) coupled with a Waters triple quadruple micromass Quattro ultima Mass Spectrometer (MS) operated in positive and negative electrospray ionization (ESI) mode was employed in analysis of the urine samples. The LC–MS/MS analysis was performed using a mobile phase made up of milli-Q- water containing 0.1% formic acid (A) and acetonitrile containing 0.1% formic acid (B) at flow rate of 0.45 ml/min and a pressure of 400 bar; the LC program is shown in Table 1 . The standards and samples (10 µl) were injected by an autosampler onto a Kinetex column (5 µm, 100 × 3 mm, 100A EVO C18). The column temperature was maintained at 40 °C. The MS parameters were: desolation temperature of 350 °C, source temperature of 120 °C and gas flow rate of 706 L/Hr. The total LC–MS/MS run time was 8 min. Qualitative and quantitative analysis was done in multiple reaction mode (MRM). Identification and confirmation of the peaks was done based on retention times and comparison of ratios of MRM transitions from the precursor ions to product ions in samples and standards.
3 Results and discussion
3.1 study population demographics and urinalysis.
Out of 100 controls who had initially been selected, 29 had abnormal urine results as shown in Table 2 , and were therefore excluded from the study. All the 41 patients who were in the inclusion criteria were included regardless of their urinalysis results because malaria poses some urinary abnormalities such as haematuria, proteinuria, urobilinogenuria and bilirubinuria [ 32 , 33 , 34 ] hence a total of 112 participants were finally included.
3.2 Infra-red (IR) spectroscopy screening of urine from patients and controls
Generally, male and female controls urine spectral profiles were similar and this agrees with the work done by Sarigul et al., [ 29 ]. The spectral profile for all controls was generally similar in the fingerprint region of 1800–600 cm −1 , variation was observed in the regions 1676–1614 cm −1 and in region 1445–1434 cm −1 where the intensities in females were higher than in males as shown in Fig. 2 . The average spectra of all patients and controls regardless of sex is shown in Fig. 3 , the profile for patients and that of controls differed at wave numbers about 3030, 1594, 1454 and 940 cm −1 . Principle component analysis (PCA) was used to determine whether patients and control urine samples could be distinguished. PCA was done on normalized data because normalization reduces variance which is not associated with spectral characteristics of samples [ 35 ]. Figure 4 is the PCA plot showing that urine from patients and controls could be distinguished. Table 3 shows the relationship between wave numbers and principal components; the observed wave numbers were assigned to functional groups based on literature [ 29 , 35 , 36 ].

Average spectra of patients and controls
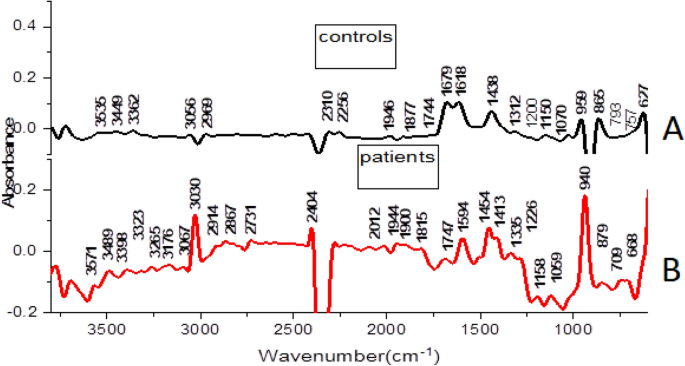
Average infra-red spectra from urine of malaria patients ( B ) and controls ( A )
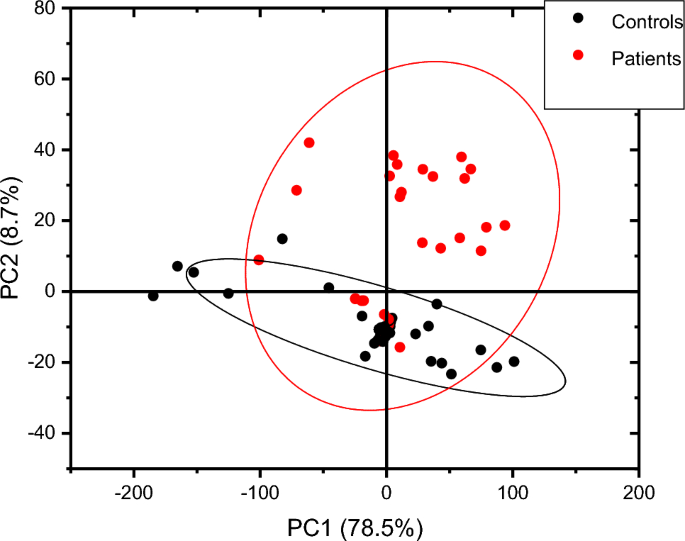
Showing score plot of controls and patients
Based on the loadings (Table 3 ), the first 3 principal components (PC) explained 90.7% of total variation. PC1 was negatively correlated to the wave numbers 1312, 2969, 3056, and 3362 cm −1 which were assigned to C–N stretching in aromatic amines, C–H and N–H stretching (Table 3 ). PC2 was positively correlated to 865, 1070, 1150, 1200, 1679 and 3449 cm −1 and also negatively correlated to 1946 cm −1 , these peaks were assigned to NH 2 , S=O, P–O bonds. PC3 was positively correlated to 1438, 1679 cm −1 and negatively correlated to 959 cm −1 and the wave numbers were assigned to C–H bending, C=N; N–H stretching and O–H bend, S–O stretch and P–OH stretching. The wave numbers were associated with amino groups, phosphates, sulphates and carboxylic acids [ 29 , 36 ] which are functional groups associated with amino acids, implying that amino acids may be perturbed during malaria infection. A common peak at about 1438 cm −1 which is associated with C–H bending in lipids was observed in all controls.
Peak at wave number 1618 cm −1 and 1679 cm −1 (related to N–H functional group) had high intensities in controls than in malaria patients. The reduction in patients may be due to amino acid metabolism during Plasmodium falciparum infection. The parasites and the infected red blood cells have limited capabilities for amino acid biosynthesis [ 38 ] and therefore utilize the host cell haemoglobin and plasma free amino acids for protein synthesis [ 36 , 37 , 38 , 39 , 40 ]; for example the parasite’s glutamate dehydrogenase utilizes glutamine as shown in equation (i) for its primary source of parasite NADPH [ 39 , 40 ];
The peak at 3030 cm −1 (C–H, NH 4 + ring stretching) had high intensity in patients compared to controls. This could be as a result of the substantial amount of ammonia produced as a catabolic by-product by Plasmodium falciparum , during malaria infection as shown in equation (i) [ 37 , 38 , 39 ] or it may be associated with amino acids. The peak at 940 cm −1 (O–H stretching of a carboxylic acid) also showed a high intensity in patients compared to controls. Parasite sequestration results into tissue hypoxia which increases the chances of anaerobic glycolysis. This could result in build-up of lactic acid leading to metabolic acidosis. Lactate clearance is decreased during malaria infection due to liver dysfunction, suppressed glycogenesis and decreased hepatic flow [ 42 ]. The wave numbers 959 cm −1 (O–H bend, S–O, P–OH), 3030 cm −1 (C–H (ring) stretching, symmetric NH 4 stretching), 1618 (C=C stretching, N–H bend (primary amine) and 1679 cm −1 (C=N stretching, C=O stretching) were responsible for differentiating controls and patients. These functional groups (phosphate, amine, carboxylic acid, sulphate,) can be found in amino acids, alluding to amino acid perturbation during Plasmodium falciparum infection.
Based on the Mann–Whitney U test, the urine spectra of controls and patients were not significantly different at a 5% level of significance (z = 0.39) perhaps because all the metabolites present in the urine of controls are also observed in urine patients although the absorbance intensity may differ. PCA showed the samples and controls in different clusters as shown in Fig. 4 , implying that intensities of the metabolites differ. Since the prominent peaks were associated with functional groups found in amino acids, samples were subjected to liquid chromatography–tandem mass spectrometry (LC–MS/MS) to establish variations in amino acid concentrations between controls and urine samples from malaria patients.
3.3 LC–MS/MS analysis of amino acid in urine samples
Five amino acids including cystine, tyrosine, histidine, lysine and creatinine were detected in the urine samples from patients and controls and were therefore quantified. Figure 5 shows the amino acids cystine, tyrosine, histidine and lysine expressed in terms of urinary creatinine while Fig. 6 shows the concentration of urinary creatinine in controls and patients urine.
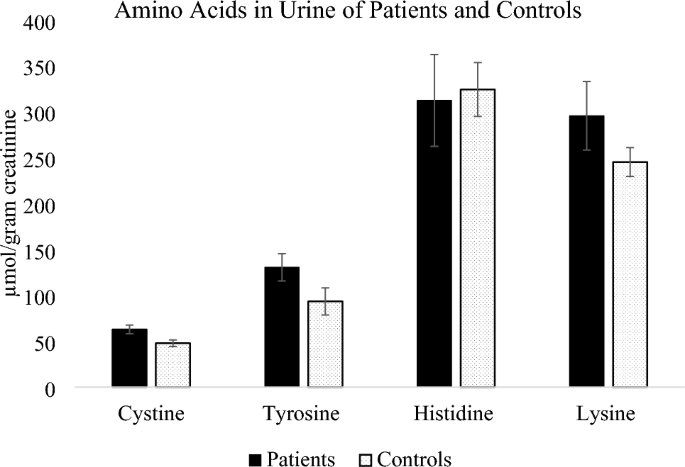
Mean values of amino acids in urine expressed in µmol/g creatinine

Mean creatinine in urine of patients and controls
Amino acids cystine, tyrosine and lysine were higher in patients compared to controls, while histidine was slightly higher in controls. The mean concentration of cystine in both patients and controls was within the normal daily excretion of cystine ranges from 0 to 100 μmol/g creatinine [ 43 ] but the mean values were significantly different (P = 0.012). In terms of parasitemia, the patients’ distribution was 36.6% mild malaria, 58.5% moderate malaria and only 4.9% severe malaria. Since severe malaria cases were few, they were considered with moderate malaria cases while assessing the influence of parasitemia on the concentration of amino acids in urine. Amino acids cystine and lysine slightly increased with parasitemia although the increase was not statistically significant as shown in Table 4 . Cystine is the predominant form of extracellular cysteine [ 44 ] and cysteine can be rapidly oxidized to produce cystine in presence of oxygen [ 45 ], the cystine/cysteine redox cycle is major regulator of cell survival [ 46 ].
Higher cysteine concentrations in P. falciparum malaria patients has been previously reported in plasma [ 47 ] with the level of cysteine found to positively correlate to malaria parasitemia in previous studies [ 48 ], thus infection with P. falciparum can be associated with increased cystine/cysteine in human urine.
Tyrosine was also higher in patients compared to controls although the difference between the means was not statistically significant (P = 0.091). Higher values of tyrosine in malaria was previously reported by Conroy et al. [ 49 ] where plasma tyrosine levels were found to increase significantly during falciparum malaria in children. Tyrosine is primarily metabolized in the liver and its metabolism might be altered since malaria results in liver damage when sporozoites infect liver cells [ 50 ]. Lysine was higher in patients compared to controls although the difference between the mean values of patients and controls did not differ significantly (P = 0.168). Lysine was reported to increase in sera of mice infected with Plasmodium berghei [ 16 ], thus dysregulation of lysine can be associated with plasmodium infection.
Histidine average amounts in urine of patients was lower than in controls although the means did not differ significantly (P = 0.827). Elevated levels of Plasmodium falciparum histidine rich protein-2 (pfHRP2) were reported in severe Plasmodium falciparum in blood [ 51 ], thus the pfHRP2 is usually used as a diagnostic marker for malaria in blood, however in our study amino acid histidine in patients was lower than in controls.
Creatinine in urine of malaria patients was higher than that in controls as shown in Fig. 6 however the means of creatinine from patients and controls were not significantly different (P = 0.85). Increased serum creatinine levels in mild and severe malaria was reported for patients [ 49 , 50 , 52 , 53 ] and has been described as among useful biochemical indicators to evaluate prognosis for malaria because patients with a high level of creatinine needed a longer duration for parasite clearance [ 54 ]. Elevated creatinine due to malaria infection was for Plasmodium vivax malaria showing that malaria infection affects renal function [ 55 ].
4 Conclusions and recommendations
This study evaluated the potential of using Infrared spectroscopy (IR) coupled with principal component analysis (PCA) in distinguishing between urine samples from people infected with malaria and controls. PCA scatter plots demonstrated a difference in clustering of patients’ urine and controls therefore IR-coupled with chemometry can potentially be applied in screening for malaria. A validation using LC–MS/MS study revealed dysregulation of amino acid cystine and an increase in creatinine alluding to potential renal injury as a result of malaria infection. The difference in amino acid concentration was observed even for mild to moderate malaria (< 100–9999 parasites/µl of blood)[ 12 ].Therefore, IR enabled identification of functional groups assigned to amino acids implying that malaria affects amino acid metabolism. This study was limited by few severe malaria cases and it was a case control study. In future metabolite finger printing with a higher resolution instrument can be done of all metabolites in the urine of patients and controls. A longitudinal study to assess the changes in the entire metabolite profile and how it varies with parasitemia during infection and after treatment can contribute more useful information.
Data availability
Data that support the findings of this study are within in text and in the supplementary file. Data on participants identity can be shared in coded formats to protect their identities on request from the corresponding author, using email [email protected].
Hasselquist D, Hellgren O, Krizanauskiene A, Pe J, Bensch S. What are malaria parasites ? 2005; 21(5): 209–211. https://doi.org/10.1016/j.pt.2005.03.007 .
Pance A. Diversify and conquer: the vaccine escapism of Plasmodium falciparum . Microorganisms. 2020;8(11):1–21. https://doi.org/10.3390/microorganisms8111748 .
Article Google Scholar
Enato EFO, Okhamafe AO. Plasmodium falciparum malaria and antimalarial interventions in sub-Saharan Africa: challenges and opportunities. Afr J Biotechnol SPEC ISS. 2005;4(13):1598–605. https://doi.org/10.4314/ajfand.v4i13.71774 .
World Health Organization. World malaria World malaria report report. World Health Organization; 2023.
Google Scholar
Baba E, et al. Effectiveness of seasonal malaria chemoprevention at scale in west and central Africa: an observational study. Lancet. 2020;396(10265):1829–40. https://doi.org/10.1016/S0140-6736(20)32227-3 .
Zimmerman PA, Howes RE. Malaria diagnosis for malaria elimination. Curr Opin Infect Dis. 2015;28(5):446–54. https://doi.org/10.1097/QCO.0000000000000191 .
Conteh L, Shuford K, Agboraw E, Kont M, Kolaczinski J. Costs and cost-effectiveness of malaria control interventions: a systematic literature review. Value Heal. 2021;24(8):1213–22. https://doi.org/10.1016/j.jval.2021.01.013 .
Satimai W, et al. Artemisinin resistance containment project in Thailand II: responses to mefloquine-artesunate combination therapy among falciparum malaria patients in provinces bordering Cambodia. Malaria J. 2012;11:1–13.
Plewes K, Leopold SJ, Kingston HWF, Dondorp AM. Malaria: what’s new in the management of malaria? Infect Dis Clin North Am. 2019;33(1):39–60. https://doi.org/10.1016/j.idc.2018.10.002 .
Wongsrichanalai C, Barcus MJ, Muth S, Sutamihardja A, Wernsdorfer WH. A review of malaria diagnostic tools: microscopy and rapid diagnostic test (RDT). Am J Trop Med Hyg. 2007;77(SUPPL. 6):119–27. https://doi.org/10.4269/ajtmh.2007.77.119 .
Abdelrazig S, et al. A metabolomic analytical approach permits identification of urinary biomarkers for Plasmodium falciparum infection: a case-control study. Malar J. 2017. https://doi.org/10.1186/s12936-017-1875-z .
Aninagyei E, Abraham J, Atiiga P, Antwi SD, Bamfo S, Acheampong DO. Evaluating the potential of using urine and saliva specimens for malaria diagnosis in suspected patients in Ghana. Malar J. 2020;19(1):1–9. https://doi.org/10.1186/s12936-020-03427-x .
Hazra RK. Overview of current diagnostic methods for malaria. Le Infez Med. 2018; 3: 199–203. https://pdfs.semanticscholar.org/6768/3e09628bbd02bf65663b033cd158676cbce7.pdf
Owusu EDA, Campillo A, Daily J, Ding XC. Acceptance and perceived value of non-invasive malaria diagnostic tests in malaria-endemic countries. Malar J. 2021;20(1):1–10. https://doi.org/10.1186/s12936-021-03911-y .
Rebelo M, Grenho R, Orban A, Hänscheid T. Transdermal diagnosis of malaria using vapor nanobubbles. Emerg Infect Dis. 2016;22(2):343–4. https://doi.org/10.3201/eid2202.151203 .
Basant A, Rege M, Sharma S, Sonawat HM. Alterations in urine, serum and brain metabolomic profiles exhibit sexual dimorphism during malaria disease progression. Malar J. 2010;9:1–13.
Trampuz A, Jereb M, Muzlovic I, Prabhu RM. Clinical review: severe malaria. Crit Care. 2003;7(4):315–23. https://doi.org/10.1186/cc2183 .
Zhang A, Sun H, Wu X, Wang X. Urine metabolomics. Clin Chim Acta. 2012;414:65–9. https://doi.org/10.1016/j.cca.2012.08.016 .
Miller IJ, Peters SR, Overmyer KA, Paulson BR, Westphall MS, Coon JJ. Real-time health monitoring through urine metabolomics. NPJ Dig Med. 2019. https://doi.org/10.1038/s41746-019-0185-y .
Bouatra S, et al. The human urine metabolome. PLoS ONE. 2013. https://doi.org/10.1371/journal.pone.0073076 .
Smolders R, Schramm KW, Nickmilder M, Schoeters G. Applicability of non-invasively collected matrices for human biomonitoring. Environ Heal A Glob Access Sci Source. 2009;8(1):1–10. https://doi.org/10.1186/1476-069X-8-8 .
Qiu S, et al. Small molecule metabolites: discovery of biomarkers and therapeutic targets. Signal Transduct Target Ther. 2023;8(1):1–37. https://doi.org/10.1038/s41392-023-01399-3 .
Waybright TJ, Van QN, Muschik GM, Conrads TP, Veenstra TD, Issaq HJ. LC-MS in metabonomics: optimization of experimental conditions for the analysis of metabolites in human urine. J Liq Chromatogr Relat Technol. 2006;29(17):2475–97. https://doi.org/10.1080/10826070600914638 .
Zhu J, et al. Urine based near-infrared spectroscopy analysis reveals a noninvasive and convenient diagnosis method for cancers: a pilot study. PeerJ. 2023;11:1–16. https://doi.org/10.7717/peerj.15895 .
Oliver KV, Vilasi A, Maréchal A, Moochhala SH, Unwin RJ, Rich PR. Infrared vibrational spectroscopy: A rapid and novel diagnostic and monitoring tool for cystinuria. Sci Rep. 2016. https://doi.org/10.1038/srep34737 .
Ramirez CAM, et al. Infrared spectroscopy of urine for the non-invasive detection of endometrial cancer. Cancers (Basel). 2022. https://doi.org/10.3390/cancers14205015 .
Goh B, et al. The application of spectroscopy techniques for diagnosis of malaria parasites and arboviruses and surveillance of mosquito vectors: a systematic review and critical appraisal of evidence. PLoS Negl Trop Dis. 2021;15(4):1–24. https://doi.org/10.1371/journal.pntd.0009218 .
Sikulu-Lord M, et al. Rapid and non-invasive detection of malaria parasites using near-infrared spectroscopy and machine learning. PLoS ONE. 2023. https://doi.org/10.1371/journal.pone.0289232 .
Sarigul N, Kurultak İ, Uslu Gökceoğlu A, Korkmaz F. Urine analysis using FTIR spectroscopy: a study on healthy adults and children. J Biophotonics. 2021. https://doi.org/10.1002/jbio.202100009 .
UNCST. National guidelines for research involving humans as research participants. Gov. Uganda; 2014. p. 1–60.
Khamis MM, Adamko DJ, El-Aneed A. Mass spectrometric based approaches in urine metabolomics and biomarker discovery. Mass Spectrometry Rev. 2017;36:115–34. https://doi.org/10.1002/mas.21455 .
Al-Mekhlafi HM, et al. Residual malaria in Jazan region, southwestern Saudi Arabia: the situation, challenges and climatic drivers of autochthonous malaria. Malar J. 2021;20(1):1–14. https://doi.org/10.1186/s12936-021-03846-4 .
Article MathSciNet Google Scholar
Ugwuja EI, Ugwu NC. Abnormal findings on dipstick urinalysis of out-patients with malaria in Abakaliki, Nigeria. J Vector Borne Dis. 2011;48(4):205–9.
da Silva JGB, Pinto JR, Barros EJG, Farias GMN, Daher EDF. Kidney involvement in malaria: an update. Rev Inst Med Trop Sao Paulo. 2017;59: e53. https://doi.org/10.1590/S1678-9946201759053 .
Mishra P, et al. At-line and inline prediction of droplet size in mayonnaise with near-infrared spectroscopy. Infrared Phys Technol. 2022;123: 104155.
Coates J. Infrared spectral interpretation: a systematic approach. Infrared Spectr Interpret A Syst Approach. 2018. https://doi.org/10.1201/9780203750841 .
Sarigul N, Korkmaz F, Kurultak I. A new artificial urine protocol to better imitate human urine. Sci Rep. 2019. https://doi.org/10.1038/s41598-019-56693-4 .
Sherman IW. Amino acid metabolism and protein synthesis in malarial parasites. Bull World Health Organ. 1977;55(2–3):265–76.
Rubach MP, et al. Kinetic and cross-sectional studies on the genesis of hypoargininemia in severe pediatric Plasmodium falciparum malaria. Infect Immun. 2019. https://doi.org/10.1128/IAI.00655-18 .
Kimoloi S, Rashid K. Potential role of Plasmodium falciparum -derived ammonia in the pathogenesis of cerebral malaria. Front Neurosci. 2015;9:1–12. https://doi.org/10.3389/fnins.2015.00234 .
Zeuthen T, et al. Ammonia permeability of the aquaglyceroporins from Plasmodium falciparum , Toxoplasma gondii and Trypansoma brucei . Mol Microbiol. 2006;61(6):1598–608. https://doi.org/10.1111/j.1365-2958.2006.05325.x .
Na J, et al. Discovery of metabolic alterations in the serum of patients infected with Plasmodium spp. by high-resolution metabolomics. Metabolomics. 2020. https://doi.org/10.1007/s11306-019-1630-2 .
Guillén M, Corella D, Cabello ML, García AM, Hernández-Yago J. Reference values of urinary excretion of cystine and dibasic aminoacids: classification of patients with cystinuria in the Valencian Community, Spain. Clin Biochem. 1999;32(1):25–30. https://doi.org/10.1016/S0009-9120(98)00087-3 .
Combs JA, Denicola GM. The non-essential amino acid cysteine becomes essential for tumor proliferation and survival. Cancers (Basel). 2019. https://doi.org/10.3390/cancers11050678 .
Baart GJE, et al. Modeling Neisseria meningitidis metabolism: from genome to metabolic fluxes. Genome Biol. 2007. https://doi.org/10.1186/gb-2007-8-7-r136 .
Banjac A, et al. The cystine/cysteine cycle: a redox cycle regulating susceptibility versus resistance to cell death. Oncogene. 2008;27(11):1618–28. https://doi.org/10.1038/sj.onc.1210796 .
Chillemi R, Zappacosta B, Simporè J, Persichilli S, Musumeci M, Musumeci S. Hyperhomocysteinemia in acute Plasmodium falciparum malaria: an effect of host-parasite interaction. Clin Chim Acta. 2004;348(1–2):113–20. https://doi.org/10.1016/j.cccn.2004.05.007 .
Besouw M, Masereeuw R, Van Den Heuvel L, Levtchenko E. Cysteamine: an old drug with new potential. Drug Discov Today. 2013;18(15–16):785–92. https://doi.org/10.1016/j.drudis.2013.02.003 .
Conroy AL, et al. Plasma amino acid concentrations in children with severe malaria are associated with mortality and worse long-term kidney and cognitive outcomes. J Infect Dis. 2022;226(12):2215–25. https://doi.org/10.1093/infdis/jiac392 .
Al-Salahy M, Shnawa B, Abed G, Mandour A, Al-Ezzi A. Parasitaemia and its relation to hematological parameters and liver function among patients malaria in Abs, Hajjah, Northwest Yemen. Interdiscip Perspect Infect Dis. 2016;2016:5954394. https://doi.org/10.1155/2016/5954394 .
Kwak JD, Young JJ, Stuij AC, Koelewijn R, van Hellemond JJ, van Genderen PJJ. A comparative study of Plasmodium falciparum histidine-rich protein 2 (PfHRP2) blood levels and peripheral blood parasitemia as parameters of disease severity in individuals with imported falciparum malaria. Travel Med Infect Dis. 2021;42: 102076. https://doi.org/10.1016/j.tmaid.2021.102076 .
Onyeneke N, Oghenejode EC, Alumonah AM, Okwonko EO, Okpogba CJ. Serum urea and creatinine in Nigerian human patients. Glob J Med Sci. 2003;2:103–6.
Aich T. P . falciparum malaria induced renal impairment. J Med Res. 2019;5(6):220–3. https://doi.org/10.31254/jmr.2019.5605 .
Bi D, et al. Biochemical characteristics of patients with imported malaria. Front Cell Infect Microbiol. 2022;12(November):1–10. https://doi.org/10.3389/fcimb.2022.1008430 .
Cruz LAB, Barral-Netto M, Andrade BB. Distinct inflammatory profile underlies pathological increases in creatinine levels associated with Plasmodium vivax malaria clinical severity. PLoS Negl Trop Dis. 2018;12(3):1–14. https://doi.org/10.1371/journal.pntd.0006306 .
Download references
Acknowledgements
The authors acknowledge funding from The Academy of Science in the Developing World TWAS Research Grant Award_21-264 RG/CHE/AF/AC_I-FR324031950.
Author information
Authors and affiliations.
Department of Chemistry, Faculty of Science, Mbarara University of Science and Technology, Plot 8-18 Mbarara Kabale Road, P.O Box 1410, Mbarara, Uganda
Grace Birungi, Joan Beryl Achar & Denis Byamugisha
You can also search for this author in PubMed Google Scholar
Contributions
This work was conceptualized by Grace Birungi. Laboratory work and manuscript writing were done by Joan Beryl Achar, Denis Byamugisha and Grace Birungi.
Corresponding author
Correspondence to Grace Birungi .
Ethics declarations
Competing interests.
Authors declare no competing interests.
Additional information
Publisher's note.
Springer Nature remains neutral with regard to jurisdictional claims in published maps and institutional affiliations.
Supplementary Information
Below is the link to the electronic supplementary material.
Supplementary Material 1.
Rights and permissions.
Open Access This article is licensed under a Creative Commons Attribution 4.0 International License, which permits use, sharing, adaptation, distribution and reproduction in any medium or format, as long as you give appropriate credit to the original author(s) and the source, provide a link to the Creative Commons licence, and indicate if changes were made. The images or other third party material in this article are included in the article's Creative Commons licence, unless indicated otherwise in a credit line to the material. If material is not included in the article's Creative Commons licence and your intended use is not permitted by statutory regulation or exceeds the permitted use, you will need to obtain permission directly from the copyright holder. To view a copy of this licence, visit http://creativecommons.org/licenses/by/4.0/ .
Reprints and permissions
About this article
Birungi, G., Achar, J.B. & Byamugisha, D. Characterization of urinary metabolites associated with malaria infection using infra-red spectroscopy and liquid chromatography–mass spectrometry in South Western Uganda. Discov Appl Sci 6 , 356 (2024). https://doi.org/10.1007/s42452-024-06061-3
Download citation
Received : 12 April 2024
Accepted : 24 June 2024
Published : 28 June 2024
DOI : https://doi.org/10.1007/s42452-024-06061-3
Share this article
Anyone you share the following link with will be able to read this content:
Sorry, a shareable link is not currently available for this article.
Provided by the Springer Nature SharedIt content-sharing initiative
- Non-invasive diagnosis
- Infra-red spectroscopy
- Liquid chromatography–mass spectrometry
- Human urine
- Find a journal
- Publish with us
- Track your research

An official website of the United States government
The .gov means it’s official. Federal government websites often end in .gov or .mil. Before sharing sensitive information, make sure you’re on a federal government site.
The site is secure. The https:// ensures that you are connecting to the official website and that any information you provide is encrypted and transmitted securely.
- Publications
- Account settings
Preview improvements coming to the PMC website in October 2024. Learn More or Try it out now .
- Advanced Search
- Journal List
- Iran J Parasitol
- v.13(1); Jan-Mar 2018
Malaria Vaccine Development: The Need for Novel Approaches: A Review Article
Shima mahmoudi.
1. Pediatric Infectious Disease Research Center, Tehran University of Medical Sciences, Tehran, Iran
2. Center for Research of Endemic Parasites of Iran (CREPI), Tehran University of Medical Sciences, Tehran, Iran
Hossein KESHAVARZ
3. Dept. of Medical Parasitology and Mycology, School of Public Health, Tehran University of Medical Sciences, Tehran, Iran
Background:
Although rigorous efforts have substantially decreased the malaria burden through decades, it still threatens the lives of millions of children. Development of an effective vaccine can provide important approach in malaria control strategies. Unfortunately, development of an effective vaccine for falciparum malaria has been hindered by the extreme complexity of malaria parasite biology, complex and diverse parasite genomes, and immune evasion by the parasites as well as the intricate nature of the parasites infection cycle. The aim of this review was to discuss the different approaches to malaria vaccine development until now.
Scientific databases, including MEDLINE (via PubMed) and SCOPUS were searched up to 30 Jan 2017 and the articles regarding malaria vaccine development were taken into examination.
Several strategies for malaria vaccine development including pre-erythrocytic vaccines, antibody-based subunit vaccines, vectored vaccines, whole sporozoite vaccines, genetically Attenuated parasites and sporozoite subunit vaccine, erythrocytic vaccines, sexual stage vaccine, transmission-blocking vaccine as well as synthetic peptides and conjugate vaccine has been introduced. However, the success has been limited thus far.
Conclusion:
Although development of malaria vaccine over the past 70 year has been continued, the discovery, development, and licensing of a malaria vaccine formulation, which meets safety, affordability, accessibility, applicability, and efficacy has not yet been achieved.
Introduction
Malaria remains as one of the leading causes of morbidity and mortality, especially in the tropics. Although rigorous efforts have substantially decreased the malaria burden through decades, it still threatens the lives of millions of children ( 1 ).
In 2015, 212 million people developed clinical malaria infection, mostly in resource-poor tropical areas of the world and in particular sub-Saharan Africa and 428000 people died of the disease ( 2 ). Of those infected, around 500000 succumb to malaria, with children under the age of 5 and pregnant women being the most susceptible.
The necessity to develop a vaccine against malaria has been emphasized from the identification of the parasite in 1897. In 1897, Ronald Ross discovered the mosquito (vectors) that transmit the disease. Further, only the female Anopheles mosquito can transmit the parasite. However, aiming to develop a highly effective malaria vaccine has led to the use wide range of new approaches ( 3 ). The emergence of resistant parasites and vectors has caused to concentrate on other controls achievements including vaccine ( 4 ).
Development of an effective vaccine can provide important approach in malaria control strategies ( 5 ). Unfortunately, development of an effective vaccine for falciparum malaria has been hindered by the extreme complexity of malaria parasite biology, complex and diverse parasite genomes, and immune evasion by the parasites as well as the intricate nature of the parasites infection cycle.
Generally, the majority of the available vaccines are divided into following categories: attenuated versions of pathogen microbes, killed microbes or protein subunits or conjugate vaccines ( 3 ).
Although vaccination has several successful strategies in reducing of some diseases incidence, development of vaccines for malaria has remained a challenge because of antigenic variability and the requirement of T-cell immunity for protection ( 5 ). Parasite vaccines usually face the challenges. They generate low immunity and they mostly need to proper adjuvants and most of the selected malaria antigens as vaccine candidates show significant genetic polymorphism they are the targets of natural immunity ( 3 ).
There has been considerable progress in development of malaria vaccines. Several factors should be considered for development of each new vaccine: pathogen life cycle and epidemiology, immune control and evasion, antigen candidate and vaccine formulation and preclinical/clinical results ( 6 ). One of the major obstacles to vaccine development is complex life-cycle of the parasite and the variability of antigens within each stage ( 5 ).
There are several malaria vaccine candidates which were undergone different phase of clinical trials; however, until now there was not a good candidate with appropriate efficacy. Because the parasite has three different life stages, there are three distinct vaccines approaches based on sporozoites, sexual and asexual forms.
In this review, we discuss the different approach of the malaria vaccine development until now. This review of the malaria literature highlights current approach of the current malaria vaccine development and discusses their status and challenges.
Scientific databases, including MEDLINE (via PubMed) and SCOPUS were searched up to 30 January 2017. There was no beginning data limitation and the articles regarding malaria vaccine development were taken into examination.
Results and Discussion Plasmodium parasites life-cycle
The three stages in the Plasmodium life cycle can be divided into two distinct categories: in the pre-erythrocytic and erythrocytic asexual and gametocytes reproduction occurs in the intermediate host’s body, and in the sexual stage sexual reproduction occurs in the mosquito vector gut ( 7 ).
Sporozoites of Plasmodium are inoculated to the subcutaneous of humans by the bite of infected Anopheles mosquitoes. After minutes, they enter to liver via bloodstream. Then, after 6–15 d tissue merozoites enter bloodstream and begin asexual blood schizogony as well as gametocyte production. After that, the gametocytes enter the midgut of vector mosquitoes and finally, sporozoites are formed due to the sexual stage of parasite development. In each stage of Plasmodium life cycle, various antigens can be introduced into the human body and they can stimulate host immune system ( 8 ).
The pre-erythrocytic, erythrocytic stages and transmission-blocking vaccines (TBVs) against asexual stages are considered as the main targets of P. falciparum parasites for vaccine development. However, recently, the old approach of whole parasite vaccination has improved rapidly ( Fig.1 ).

Life cycle of the malaria parasite and breaking of the cycle by major vaccine groups
*Discontinued/inactive
Immunity against the Malaria Parasite
Numerous innate and adaptive immune mechanisms are involved for control and elimination of malaria due to infecting of different cell types and wide range of its localizations ( 9 ). Understanding the immune responses to the malaria parasites is considered as the crucial goal for development of vaccines.
Protective immunity against malaria requires interaction between both innate and adaptive immunity (dendritic cells, NK cells, B cells, CD4 + and CD8 + T cells). On the other hand, to avoid being eliminated by the host immunity, the parasite introduce many escape strategies ( 9 , 10 ) ( Table 1 ).
Parasite immune escape strategies
Prevent parasite elimination, inhibiting phagocyte function and reducing phagocyte numbers |
Decrease DC functions |
Antigenic diversion |
Epitope masking |
Avoid recognition by antibody |
Prevent the action of neutralizing antibodies |
Poor or limited B-cell memory |
Poor or no antibody response by inducing immunological tolerance |
Avoid T-cell recognition |
Poor T-cell response |
Anergy and/or T-cell exhaustion |
Negative regulation of immune responses |
Production of suppressor factors |
Smoke-Screen strategy by cross-reactivity |
Homologies with Host Proteins |
Pre-erythrocytic (PE) vaccines Radiation-attenuated sporozoites
Development of modern malaria vaccine stems from rodents, primates, and human volunteers immunization with irradiated sporozoites since 1960s ( 11 , 12 ).
In 1967, immunization of mice was showed with radiation-attenuated Plasmodium berghei sporozoites ( 11 ). In 2002, the gamma radiation-attenuated sporozoites inside infected Anopheles mosquitoes lead to a complete protection in human. This irradiation approach had two major flaws: it was not cost-effective and not practical on a large scale ( 13 ).
Monoclonal antibodies and recombinant DNA methodologies
In the early 1980s, technologies such as monoclonal antibodies and recombinant DNA methodologies were introduced. Although several antigens had been cloned and sequenced ( 14 , 15 ), their role in protective immunity was only assessed by testing homologues in experimental infections.
Antibody-based subunit vaccines
A drawback of this method is the lack of inflammatory cytokines induced by most subunit vaccine the need for adjuvant for immunogenicity and these vaccines could not target the liver-stage malaria parasite. In the future, several factors including evaluation of additional targets may increase the efficacy of antibody-based subunit vaccines such as PfCSP. In addition, finding of both PfCSP and non-PfCSP alike antigens for capturing of natural parasite heterogeneity and developing vaccines which induce long-lasting protection should be considered ( 16 ).
PE vaccine could prevent invasion of hepatocytes by sporozoite or kill parasites within infected hepatocytes. Efforts to identify novel sporozoite antibody targets have been difficult. Novel antigen selection will need to draw and combinations of multiple antigens may act synergistically to improve protection.
“RTS, S/AS01”, a subunit vaccine, elicit CD4 T cell and antibody responses to the P. falciparum circumsporozoite protein, or PfCSP ( 17 ). Although this vaccine has been recommended for licensure by EMEA and is the first vaccine to undergo large-scale phase 3 evaluations in seven African countries, it's efficacy in infants is relatively low, and the vaccine apparently will not meet the goal of malaria eradication by itself ( 18 ).
Vectored vaccines
Vectored vaccines were introduced in attempts to induce higher efficacy. Chimpanzee adenoviruses (ChAds) encoding the thrombospondin-related adhesion protein (TRAP) pre-erythrocytic antigen was used to prime an immune response and then it was boosted by another viral vector, modified vaccinia virus Ankara (MVA) encoding the same TRAP insert ( 19 , 20 ). This particular prime-boost approach first discovered in malaria introduces much higher T-cell responses than single vector immunization.
Whole sporozoite vaccines
Most recently, the old approach of whole parasite vaccination has progressed rapidly through three major technologies including Radiation-attenuated P. falciparum sporozoites (RAS), Chloroquine Prophylaxis and Sporozoites (CPS) and Genetically Attenuated Parasites (GAP) ( 21 ).
Three different strategies have been employed to induce protective responses against liver stages. Irradiated sporozoites have the ability to invade liver cells and initiating liver stage development including expression of new proteins. They do not undergo nuclear division and formation of liver stage merozoites ( 22 ). It is more possible that PfSPZ-based vaccines will be introduced in WHO malaria vaccine roadmap during the next two to three years ( 23 ).
Genetically Attenuated Parasites (GAP)
GAPs are generated by gene deletions, genome edition or stage-specific toxins expression ( 21 ).
Deletion of two pre-erythrocytic genes (p52-/p36-) in the NF54 strain resulted in introducing of the first generation P. falciparum GAP ( 24 ). The first experiments in mice demonstrated that single gene deletions using p52-/p36 genetically attenuated parasites (GAP) could elicit long-lasting protection mediated by CD8+ T cells ( 23 , 24 ). GAP parasites induced larger and broader CD8+ T cell responses and long-lasting effector memory CD8+ T cells than RAS parasites in mice ( 25 , 26 ).
Recently, triple KO Pf GAP (p52-/p36-/SAP-) ( 27 ) and alternative P. falciparum GAP vaccine is based on b9/slarp deficient sporozoites ( 28 ) was introduced. RAS Sanaria® PfSPZ-GA1 is another genetically attenuated strain, with deletions of the b9 and slarp genes ( 28 ). GAP parasites recognize antigens in both the late liver stages and blood and elicit stage transcending immunity. This may lead to identification of novel approach for development of a multi-stage subunit vaccine ( 29 ).
Chloroquine Prophylaxis and Sporozoites (CPS)
Another approach for human immunization with whole sporozoites combines the bites of non-attenuated fully infectious P. falciparum -infected mosquitoes with chloroquine prophylaxis (CPS) ( 30 ). Chloroquine prevent blood stage development, while it has no effect on sporozoites and liver stages ( 31 ). The CPS procedure is safe and well tolerated and requires for fewer bites of fully infective mosquitoes to elicit 100% efficacy compared to RAS immunization.
The probable reasons for its higher efficacy could be due to expression of more liver stage antigens and development of larger antigen loads than RAS. In addition, the rout of immunization by bite might induce more potent immune responses than IV immunization with P. falciparum RAS ( 32 )
The PfSPZ Vaccine approach has been adapted for CPS. In addition, nonirradiated sporozoites can be administered with chloroquine prophylaxis (called Sanaria® PfSPZ-CVac) ( 23 ).
Sporozoite subunit vaccination
The circumsporozoite protein (CSP), a sporozoite surface antigen, was introduced in the era of subunit vaccine development for malaria ( 12 ).
Although subunit vaccines can eliminate the risk of reversion and have good safety compared to inactivated or attenuated pathogen vaccines, they have weakness in immune stimulation. Nanoparticles are introduced in the era of subunit vaccine development and they can enhance the efficacy of these vaccines by extending of the antigen release and circulation time ( 33 ).
However, production of a full-length CSP was unsuccessful, several substitute constructs such as R16tet32, R32tet32, R48tet32, and R32LR were produced ( 34 ). The RTS, S vaccine is another subunit vaccine based on the CSP repeat and C-terminal regions formulated with hepatitis C surface antigen in AS adjuvants ( 23 ).
Erythrocytic vaccines
Erythrocytic or blood-stage vaccines ( Fig.1 ), aim to stop the rapid invasion and asexual reproduction of the parasite in the red blood cells. Effective vaccination with blood stage would prevent complications such as renal failure and all manifestation of severe malaria in pregnancy. P. falciparum erythrocyte membrane protein 1 (PfEMP1), expressed on the surface of infected erythrocytes, was first cloned in 1995 and was considered as the prime target for an anti-complication vaccine.
At present, several clinical trials on blood-stage antigens including apical membrane antigen 1 (AMA1), erythrocyte-binding antigen-175 (EBA-175), glutamate-rich protein (GLURP), merozoite surface protein (MSP) 1, MSP2 and MSP3 and serine repeat antigen 5 (SERA5) have performed ( 35 ). Until now, two phases II clinical trial have been conducted with PfAMA-1. One of these trials, AMA1 vaccine with AS02 adjuvant was applied in 383 Malian children. Another vaccine trial with AMA-13D7 and AMA1-FVO and ALhydrogel in 279 children was studied; however, no significant impact on clinical malaria episodes was found ( 36 ). Although MSP1 vaccine could produce protective response s in Monkeys, the Trial phase II this vaccine with AS02 adjuvant in Kenyan children indicated no significant impact on clinical malaria ( 37 ).
The possible reason might be due to the extremely polymorphic nature of the vaccine structures ( 38 – 48 ). The combination malaria vaccine consists MSP-1, MSP-2 and RESA antigens with Montanide adjuvant completed phase 2 trial on 120 children 5–9 yr old. The vaccine showed no efficacy against clinical malaria ( 4 ).
None of the assays/models has been proven as a surrogate of protection and no blood-stage vaccines have shown strong efficacy in a large phase II (or III) trial. Therefore, discovery of novel antigen is highly recommended.
Sexual stage vaccine
Only a few sexual stage vaccines in preclinical studies were introduced and only phase 1 trial is underway. This vaccine is intended to block the life-cycle of Anopheles mosquitoes.
Previous clinical trials of sexual stage vaccines involved ookinete antigens Pfs25 from P. falciparum and Pvs25 from P. vivax . However, these studies were not successful due to unacceptable reactogenicity most likely related to the adjuvant ( 49 ).
Transmission-blocking vaccine (TBV)
TBVs is an indirect approach to a vaccine and target sexual stage antigens of Plasmodium ( 50 ). One TBV candidate vaccine is the Pfs 25-EPA. Evidence shows that Pfs48/45 is a good TBV candidate ( 50 ); however, it's development have several challenges such as high epitopes variability for protective antibodies and the appropriate folded recombinant protein and improper secondary structure due to incorrect cysteine connectivity ( 50 ).
Combination vaccines
Since developing of highly effective vaccines based on any single life cycle stage was not successful, introducing of effective vaccines which include antigens from more than one stage of the parasite's life cycle is highly suggested ( 3 ).
Several antigens including Py and Pb antigens alone or in combination with CSP have been selected for combination vaccine studies. It has been reported that PyLISP1 and PySLARP, PbLISP1, PbSLARP and PbPF3D7conferred significantly greater protection than that seen with CSP ( 51 ).
Synthetic peptides and conjugate vaccine
Synthetic peptides have been exploited also as an approach for the development of a malaria vaccine. SPf66, a peptide-based candidate vaccine containing antigens from the blood stages of malaria linked together with an antigen from the sporozoite stage, and is targeted mainly against the blood (asexual) stages showed apparent efficacy in new world monkeys and humans ( 52 ); however, independent field efficacy trials in Africa and Asia failed to demonstrate good protection.
Another synthetic peptide candidates vaccine derived from two P. falciparum blood-stage antigens, MSP-1 and MSP-2, was introduced in Phase I studies in Sri Lanka, but it was in doubt that it was able to induce significant levels of antibodies against the parent blood-stage epitopes and fixed merozoites or not ( 53 ).
Synthetic vaccine based on peptide-tetanus toxoid conjugated was introduced ( 53 ). Due to the low potency of first-generation DNA vaccines ( 54 ), heterologous prime-boost immunization approaches with non-replicating viral vectors have been exploited ( 55 ).
Other approaches
Parasite toxins (GPI-based) and adhesion ligands such as PfEMP1 are considered as another approach to vaccine development. Use of parasite antigens, such as the Var2 protein preferentially expressed in the placenta is another vaccine development method to prevent malaria in pregnancy.
The development of passive immunization by gene therapy could be considered as another potential promise particularly for pathogens which can evade from current vaccination strategies due to antigenic variability ( 56 ).
Vectored immunoprophylaxis has been demonstrated as another approach which was effective in a host of animal models for the prevention of malaria ( 56 ).
The march toward development of new approaches will require continued efforts and resources. Some approaches based on reverse vaccinology in order to search the genome for finding the best protective antigens is highly recommended to developing a multicomponent vaccine with full and long-lasting protection.
Since a malaria vaccine would be an important tool for prevention and treatment, further study on using of alternative parasite targets and vaccination strategies, and evaluating of other vaccine candidates in the pipeline through vaccine trials, are highly recommended.
Acknowledgments
No financial source was available for this study.
Conflict of interest
The authors declare that there is no conflict of interest.

COMMENTS
1. Introduction. Malaria affected an estimated 219 million people causing 435,000 deaths in 2017 globally. This burden of morbidity and mortality is a result of more than a century of global effort and research aimed at improving the prevention, diagnosis, and treatment of malaria [].Malaria is the most common disease in Africa and some countries in Asia with the highest number of indigenous ...
2. Materials and Methods . A systematic literature review was conducted using the PubMed online database. Review and non-review articles were identified using the following search terms, "malaria diagnosis", "malaria control and elimination diagnostics", "malaria diagnosis challenges", "saliva malaria diagnostic", "Innovative malaria tools" and "Asymptomatic malaria".
Malaria is a mosquito-transmitted infection that affects more than 200 million people worldwide, with the highest morbidity and mortality in Africa. Elimination, through vector control approaches ...
A total of 206 published malaria studies between 2013 and 2017 from the "WWARN Clinical Trials Publication Library" were selected and assessed in full-text in this review ( Figure 1 ). Of the 206 published studies, 51% (104/206) were conducted in Africa, 38% (78/206) in Asia, 8% (17/206) in the Americas, and 3% (7/206) were multiregional.
Purpose of Review Malaria is an important human parasitic disease affecting the population of tropical, subtropical regions as well as travelers to these areas. The purpose of this article is to provide clinicians practicing in non-endemic areas with a comprehensive overview of the recent data on microbiologic and pathophysiologic features of five Plasmodium parasites, clinical presentation of ...
Malaria is a mosquito-borne disease that is caused by Plasmodium parasites. Patients with malaria experience flu-like symptoms and, in severe cases, the disease can progress to neurological ...
The large number of deaths caused by malaria each year has increased interest in the development of effective malaria diagnoses. At the early-stage of infection, patients show non-specific symptoms or are asymptomatic, which makes it difficult for clinical diagnosis, especially in non-endemic areas. Alternative diagnostic methods that are timely and effective are required to identify ...
Global interest in malaria elimination has prompted research on active test and treat (TaT) strategies. A systematic review and meta-analysis were conducted to assess the effectiveness of TaT strategies to reduce malaria transmission. A total of 72 empirical research and 24 modelling studies were identified, mainly focused on proactive mass TaT (MTaT) and reactive case detection (RACD) in ...
Further understanding of the pathophysiology of malaria and the biology of the parasite will open doors to new antimalarial treatments. Plasmodium falciparum malaria, an infectious disease caused ...
Severe malaria is a medical emergency. It is a major cause of preventable childhood death in tropical countries. Severe malaria justifies considerable global investment in malaria control and elimination yet, increasingly, international agencies, funders and policy makers are unfamiliar with it, and so it is overlooked. In sub-Saharan Africa, severe malaria is overdiagnosed in clinical practice.
Introduction. Malaria is a parasitic disease, endemic in tropical and subtropical regions, with an estimated 247 million clinical cases and 619 000 deaths globally in 2021, the majority in sub-Saharan Africa (SSA) [1].In addition to the high morbidity and mortality caused by malaria, people living in endemic areas often have persistent low-level parasitaemia in absence of acute symptoms, as a ...
Background: Asymptomatic malaria infections are highly prevalent in endemic areas. Objectives: This systematic review aimed to estimate the pooled prevalence of malaria parasites in migrants screened in non-endemic areas. Data sources: MEDLINE-Ovid, EMBASE, Web of Science, Global Health, Lilacs, Cochrane, and MedRxiv. Study eligibility criteria: Cross-sectional studies and observational ...
Following unsuccessful eradication attempts there was a resurgence of malaria towards the end of the 20th century. Renewed control efforts using a range of improved tools, such as long-lasting insecticide-treated bednets and artemisinin-based combination therapies, have more than halved the global burden of disease, but it remains high with 445 000 deaths and more than 200 million cases in 2016.
Abstract. Malaria is one of the most devastating infectious diseases of humans. It is problematic clinically and economically as it prevails in poorer countries and regions, strongly hindering socioeconomic development. The causative agents of malaria are unicellular protozoan parasites belonging to the genus Plasmodium.
The objective of this study was to estimate the pooled malaria parasite prevalence in migrants residing in non-endemic countries and screened for Plasmodium parasites. Methods We carried out a systematic review and meta-analysis, designed and reported following the Meta-analysis of Observational Studies in Epidemiology guidelines [
Purpose of Review Malaria is a prevalent disease in travelers to and residents of malaria-endemic regions. Health care workers in both endemic and non-endemic settings should be familiar with the latest evidence for the diagnosis, management and prevention of malaria. This article will discuss the recent malaria epidemiologic and medical literature to review the progress, challenges, and ...
Question 2. What might next-generation malaria vaccines look like? Many next-generation malaria vaccines are currently in clinical testing ().Some use novel approaches including live attenuated sporozoite inoculations, RNA-based platforms, and a combination of existing P.falciparum CSP-based vaccines with antigens from other stages of the parasite life cycle.
Simian malaria from wild non-human primate populations is increasingly recognised as a public health threat and is now the main cause of human malaria in Malaysia and some regions of Brazil. In 2022, WHO changed malaria elimination certification guidelines to require non-negligible risks of simian malaria in people, leaving many countries with no pathway to elimination. We review the global ...
Malaria is the biggest killer among vector-borne diseases [] and has claimed the lives of milllions of people over centuries [].In 2020, 241 million cases were reported leading to 627,000 deaths. The African region has paid the highest tributes with 96% of all deaths [].Malaria disease is caused by Plasmodium parasites, which are transmitted to humans by the bites of infected female mosquitoes ...
The interaction between a pathogen and its host can lead to health or disease. Malaria is caused by different Plasmodium species, and even if most attention is directed to those species that cause human malaria, Plasmodium parasites can infect several other vertebrates, including birds. This Special Research Topic of Frontiers in Immunology brings original, mini-reviews, perspectives, and ...
1. It is an endemic vector-borne parasitic disease caused by protozoan parasites of the genus Plasmodium in. tropical and subtropical regions worldwide. 2. Plasmodium consists of over 200 species ...
2.2 Study design. A mixed method design was used to collect qualitative and quantitative data. A case-control study design was used; urine samples were collected from malaria patients (cases), who were confirmed Plasmodium falciparum positive using blood film microscopy regardless of the level of parasitemia, before treatment. Controls were sex-matched healthy people with similar age to the ...
CHAPTER 1 Literature review. 1.1 Malaria in Africa. Malaria is a common and life-threatening disease in many tropical and subtropical developing countries. There were an estimated 881 000 malaria deaths during 2006, of which 91% were in Africa and 85% were of children under 5 years of age (WHO, 2008). Malaria is responsible for one out of every ...
Severe malaria remains a burden predominantly among young children (3 to 59 months) across a wide range of community prevalence typical of East Africa. This study offers a quantitative framework for linking malaria parasite prevalence and severe disease outcomes in children. Keywords: Malaria, Plasmodium falciparum, severe malaria, anaemia ...
Unfortunately, development of an effective vaccine for falciparum malaria has been hindered by the extreme complexity of malaria parasite biology, complex and diverse parasite genomes, and immune evasion by the parasites as well as the intricate nature of the parasites infection cycle. The aim of this review was to discuss the different ...